Organic cathode materials for aqueous zinc-organic batteries
Abstract
Aqueous zinc batteries that utilize metallic Zn as the anode are considered as a promising alternative to lithium-ion batteries due to their intrinsic high safety, low cost, and relatively high energy density. Compared to inorganic cathodes, organic cathodes exhibit several advantages including high theoretical capacity, tunable structure, abundant sources, and environmental friendliness. In this paper, we summarize the recent progress in organic cathodes for aqueous zinc-organic batteries, covering the working mechanisms of three typical types of organic cathodes, their electrochemical performance, and common strategies for further improvement. Finally, we discuss the current challenges and possible future research directions. We hope this review will offer useful information for exploring high-performance organic cathodes.
Keywords
INTRODUCTION
Green energy and new energy storage technologies are vital for addressing the environmental challenges and achieving the carbon neutrality goal. Since Sony introduced the first commercial lithium-ion batteries (LIBs), they have been widely adopted across various fields owing to their high efficiency and decent lifespan[1,2]. However, they also have some drawbacks, such as the safety risks, limited lithium reserves and high cost[3,4]. As a promising alternative to LIBs, aqueous zinc-ion batteries (AZIBs) have garnered significant interest owing to their excellent theoretical capacity (820 mAh·g-1), appropriate redox potential
Cathode materials play a crucial role in the performance of AZIBs. Currently, the main types of inorganic cathode materials are V/Mn/Co/Mo-based compounds[10-13] and Prussian blue analogs[14,15]. However, these materials often suffer from slow kinetics and structural instability due to the dissolution and/or lattice evolution during the electrochemical process[16,17]. Moreover, some inorganic materials contain harmful and toxic elements that would cause negative environmental impacts. On the other hand, organic materials are more suitable for AZIB cathodes, benefiting from their high theoretical capacity, environmental friendliness, designable structure, and wide availability[18].
As research on AZIBs advances, various organic materials have been employed to develop aqueous zinc-organic batteries (AZOBs)[19-25]. However, several challenges, including the low conductivity and stability of organic materials and the unclear charge storage mechanisms, impede their further development. There is a pressing need to consolidate and delve deeper insights into these issues to devise effective solutions. In this review, we present a comprehensive overview and future outlook on AZOBs. We explore their history and mechanisms, the recent advancements in organic cathode, and strategies for enhancing their electrochemical performance. Lastly, we discuss the challenges facing organic cathodes and potential future directions.
DEVELOPMENT HISTORY AND ELECTROCHEMICAL MECHANISMS
Development history
AZOBs are composed of anodes, electrolytes, separators, and organic cathodes. The anode is mostly commercial high purity zinc foil. The electrolyte is usually a weakly acidic or neutral aqueous solution containing soluble zinc salts. The separator is generally a glass microfiber filter that separates the anode and cathode and prevents short circuits. The organic cathode is generally prepared by mixing organic materials with active functional groups, conductive agents (e.g., acetylene black and ketjen black), and binders (e.g., polyvinylidene fluoride and carboxymethylcellulose) in different proportions. The organic materials, which are abundant on earth and consist mainly of H, C, N, O, and other elements such as F and S, can be synthesized through biomass or artificial synthesis[26].
The organic cathode materials almost determine the electrochemical properties of AZOBs. In the past few decades, substantial advancements have been achieved in investigating organic cathode materials for AZOBs [Figure 1]. In 1985, Macdiarmid et al. first used organics (polyaniline) as a cathode for AZIBs[27]. Since then, researchers have reported various organic compounds with different redox-active groups as cathodes for AZOBs, such as nitronyl nitroxides[28], organosulfur compounds[29], conjugated carbonyl compounds[30], triphenylamine derivatives[31], imine compounds[32], dual redox groups compounds[33], nitroaromatics[34], and azobenzene compounds[35]. Despite these impressive achievements, designing suitable organic materials to construct AZOBs with high capacity, decent rate performance, and excellent long lifespan remains highly challenging.
Electrochemical mechanisms
Organic cathode materials of AZOBs can be categorized as p-, n-, and bipolar-type materials based on the change of the free radical state of redox-active groups[24,36,37]. Figure 2 presents the corresponding redox mechanisms. For p-type organics (e.g., nitronyl nitroxides, triphenylamine derivatives, and organosulfur compounds), they typically lose electrons and get oxidized; therefore, they must bind to anions in the electrolyte (e.g., Cl-, BF4-, SO42-, ClO4-, CF3SO3- or CH3COO-) to remain electric neutrality. On the contrary, n-type organics (such as conjugated carbonyl compounds, imine, and azo compounds) acquire electrons and convert to anions, which can then be combined with cations (e.g., Zn2+ or H+) to maintain charge balance. Bipolar-type organics exhibit both p- and n-type properties and can be either reduced or oxidized within specific voltage windows[38]. In general, p-type organics exhibit low capacities due to limited redox-active molecular structures, whereas n-type organics often possess high specific capacities[21].
Based on the different redox mechanisms, four ion storage mechanisms have been proposed for zinc-organic batteries, i.e., Zn2+ storage, H+ storage, co-storage of H+ and Zn2+, and anion storage [Figure 3]. The most reported mechanism is the Zn2+ storage. Reversible uptake/removal of Zn2+ takes place during the charging and discharging by coordination with the active center of organics. For example, the active center (C=O) of pyrene-4,5,9,10-tetraone (PTO) can obtain electrons during discharge and convert to anions
Figure 3. Schematic diagram of four different types of ion storage mechanisms for AZOBs during discharging and charging. AZOBs: Aqueous zinc-organic batteries.
The unique redox mechanism of organic electrodes makes it possible to accommodate various carriers. Compared with Zn2+, H+ with a smaller ion radius and lower atomic mass is easier to be inserted into organic cathode materials. Tie et al. first observed the reversible uptake/removal of H+ in diquinoxalino[2,3-a:2’,3’-c]phenazine (HATN) cathode[32]. During the discharge process, the C=N functional group in HATN transforms into -C-N-, combining with H+ generated by the decomposition of water in electrolyte. Conversely, -C-N- is reversibly oxidized to C=N during charging and H+ is removed from the HATN cathode. The rapid kinetics of H+ uptake/removal endow organic electrodes with excellent electrochemical performance[41,42].
Although the uptake/removal of H+ is relatively fast, Zn2+ may also participate in the storage process together since it has a much higher concentration than H+ in the electrolyte. Actually, the phenomenon of Zn2+/H+ co-storage has already been reported in many organic compounds such as dibenzo[b,i]thianthrene-5,7,12,14-tetraone (DTT)[43], diquinoxalino[2,3-a:2′,3′-c]phenazine-2,8,14-tricarbonitrile (HATN-3CN)[44], and hexaazatrinaphthalene-quione (HATNQ)[45]. For example, Wang et al. confirmed co-storage phenomenon of Zn2+/H+ in the DTT cathode[43]. Both experiments and theoretical calculations have proved that Zn2+ and H+ can insert this cathode to form DTT2(H+)4(Zn2+), improving its stability.
The anion storage mechanism usually occurs in p-type organics, which typically first lose electrons and yield cations, which then bind to anions from the electrolyte. For instance, the nitroxyl radicals of poly(2,2,6,6-tetramethylpiperidinyloxy-4-yl vinylether) (PTVE) lose electrons and are oxidized to -N+=O during charging, enabling them to bind with absorbed anions (Cl-) from electrolyte. In the process of subsequent discharge, -N+=O reversibly transforms back to nitroxyl radicals, and the anion is removed from the PTVE and released to the electrolyte[28].
DESIGN OF ORGANIC CATHODES
In the above section, we have discussed the working mechanisms of distinct types of organic cathodes. In general, the molecular structure plays an essential role in determining their redox/ion storage mechanism and, thus, the electrochemical performance. Next, we will discuss the properties and applications of these organic cathodes in AZOBs.
P-type organic cathodes
Nitronyl nitroxides
Nitronyl nitroxides typically show fast redox kinetics and high redox voltage[46]. In 2009, Koshika et al. investigated PTVE with nitroxyl radicals as a cathode for AZOBs[46], which possessed a voltage plateau of
Figure 4. (A) Cycling performance of PTVE cathode. Reproduced with permission[46]. Copyright 2009, Wiley Online Library; (B) CV profiles of Zn//PTVE battery based on 1M ZnSO4 electrolyte. Reproduced with permission[47]. Copyright 2020, Wiley Online Library; (C) Cycling performance of TEMPO cathode. Reproduced with permission[48]. Copyright 2016, Wiley Online Library; (D) CV profiles of PEDOT cathode. Reproduced with permission[50]. Copyright 2015, Wiley Online Library; (E) Rate capability of PexTTF. Reproduced with permission[29]. Copyright 2016, Springer Nature; (F) CV curves of thianthrene cathode. Reproduced with permission[51]. Copyright 2022, Wiley Online Library; (G) Diagram of the function of CNC membrane. Reproduced with permission[31]. Copyright 2019, American Chemical Society; (H) Synthesis route of m-PTPA. Reproduced with permission[52]. Copyright 2021, Wiley Online Library; (I) The relative solubility of DMPZ in different electrolyte solvents. Reproduced with permission[53]. Copyright 2022, American Chemical Society. PTVE: Poly(2,2,6,6-tetramethylpiperidinyloxy-4-yl vinylether); CV: cyclic voltammetry; TEMPO: 2,2,6,6-tetramethylpiperidinyl-N-oxyl; PEDOT: poly(3,4-ethylenedioxythiophene); PexTTF: 9,10-di(1,3-dithiol-2-ylidene)-9,10-dihydroanthracene moieties; CNC: cellulose nanocrystal; m-PTPA: porous polytriphenylamine; DMPZ: 5,10-dihydro-5,10-dimethylphenazine.
Organosulfur compounds
Organosulfur compounds are also p-type organics, with sulfur as the active site. In 1998, C´iric´-Marjanovic´ et al. prepared a rechargeable zinc battery based on polythiophene (PT) cathode[49]. In non-aqueous electrolytes, PT//Zn batteries can provide a discharge voltage of 1.25 V and a Coulombic efficiency (CE) of over 95% at low current densities (10-50 µA·cm-2). However, as the current density increased, the CE of PT//Zn batteries significantly decreased. Poly(3,4-ethylenedioxythiophene) (PEDOT) is another common conjugated sulfide compound that can be synthesized using PT. In 2015, Simons et al. designed rechargeable PEDOT//Zn batteries using imidazolium-based ionic liquid as the electrolytes[50]. The cyclic voltammetry (CV) curves are almost identical under various cycles [Figure 4D), indicating a good structural reversibility of the PEDOT cathode. Subsequently, Häupler et al. prepared a poly(acetylene)-based polymer cathode with 9,10-di(1,3-dithiol-2-ylidene)-9,10-dihydroanthracene moieties (PexTTF) for AZOBs[29]. Thanks to the conjugated skeleton that improves the electron transfer, the battery delivered a remarkable rate capacity (111 mAh·g-1 at 120 C). Additionally, it provided an 81 mAh·g-1 capacity after 9,400 cycles under 10 C, with a loss of approximately 14% [Figure 4E]. Recently, Cui et al. reported three organic sulfur compounds (thianthrene, phenoxathiine and phenothiazine) as cathodes for zinc metal batteries[51]. They found that electron cloud density is a key factor influencing the voltage of organics. Organics with a uniform distribution of electron clouds are more likely to lose electrons, leading to an increase in electron loss energy, which, in turn, provides a relatively high voltage. The discharge voltage of thianthrene with symmetrically distributed electron clouds can reach 1.5 V in a solution of ethyl methyl carbonate with 1M zinc trifluoromethanesulfonate [Zn(OTF)2] as electrolyte, which is higher than that of phenoxathiine and phenothiazine. The operating voltage can be further increased by electrolyte design by manipulating the anionic de-solvation energy. In Zn(OTF)2 electrolyte using acetonitrile as the solvent, the voltage of the thianthrene cathode can reach 1.7 V [Figure 4F].
Triphenylamine derivatives
Triphenylamine derivatives with strongly electron-donating N atoms can readily oxidize to form positively charged cationic radicals[22]. For example, the tertiary nitrogen of 1,4 bis(diphenylamino)benzene (BDB) molecule undergoes reversible oxidation/reduction in two steps, while anions are inserted/released from high concentration aqueous electrolytes with high oxidative stability[31]. However, the BDB discharge products are severely dissolved in the electrolyte, leading to a gradual loss of capacity during cycling. Glatz et al. introduced an additional cellulose membrane and a glass fiber separator to limit the dissolution of BDB discharged products [Figure 4G]. The BDB cathode combined with cellulose nanocrystal (CNC) membrane can maintain an ~82% capacity retention over 500 cycles at 0.39 A·g-1 and exhibit decent cycling life. To further enhance the stability of triphenylamine derivatives, Zhang et al. prepared a porous polytriphenylamine (m-PTPA) as the cathode of AZOBs [Figure 4H][52]. The m-PTPA with insoluble polymerization skeleton and specific 3D covalent organic framework exhibited a decent electrochemical stability (an 87.6% retention over 1,000 cycles under 16 A·g-1). In addition to adjusting the molecular structure, electrolyte design can also improve the stability of triphenylamine derivatives. Lee et al. found that the solubility of 5,10-dihydro-5,10-dimethylphenazine (DMPZ) in water is significantly lower than that in most non-proton solvents [Figure 4I][53]. A DMPZ cathode can maintain an ~81% capacity retention over 1,000 cycles at 0.255 A·g-1 in 17 m NaClO4 aqueous electrolyte, which proves the decent cycling stability.
Conductive polymers
Conductive polymers (CPs) have high conductivities and highly delocalized electron clouds, which would contribute to rapid redox kinetics[22,54]. Various polymers, such as polypyrrole (PPy)[55], polyindole (PIn)[56,57], and poly(5-cyanoindole)[58], have been reported as cathodes for AZOBs [Figure 5A]. Among them, PPy has been extensively explored benefiting from its high redox reversibility, non-toxicity, and decent conductivity. Wang et al. prepared the Zn//PPy cell based on PPy cathodes and obtained a capacity of 123 mAh·g-1 at
Figure 5. (A) The molecular structure of p-type conductive polymers, including PPy, PIn and poly(5-cyanoindole); (B) (Dis-)charge profiles of Zn//PPy battery under different currents. Reproduced with permission[55]. Copyright 2018, Royal Society of Chemistry; (C) Rate capability of the Zn//poly(5-cyanoindole) battery. Reproduced with permission[58]. Copyright 2015, Elsevier. PPy: Polypyrrole.
In summary, p-type organic cathodes generally exhibit high redox potentials, which can be attributed to the nature of their redox reactions. In p-type materials, the redox reactions involve the removal of electrons from the highest occupied molecular orbital (HOMO) or single occupied molecular orbital (SOMO) during oxidation[59]. This process typically results in higher redox potentials compared to n-type materials (which will be discussed later). However, high voltages inevitably lead to the decomposition of aqueous electrolytes, which results in poor cycling performance. Using organic electrolytes can effectively solve this problem[51,60], but it suffers from safety hazards and high costs. Introducing electrolyte additives could be a viable way to improve the stability of aqueous electrolytes as their efficacy has already been confirmed in aqueous Zn batteries[61,62]. Moreover, some p-type organic cathodes and their discharge products in aqueous electrolytes suffer from severe dissolution and thus poor cycling lifespan, which can be improved by electrolyte and separator design and the structural optimization through co-polymerization[29,48]. It is worth mentioning that most p-type organic cathodes suffer from complex preparation processes and low capacity, which hinder their application. In contrast, n-type organic cathodes often possess high capacity, and their preparation is relatively simple. Below, we will discuss the latest progress in n-type organic cathodes for AZOBs.
N-type organic cathodes
Conjugated carbonyl compounds
As a typical N-type cathode material, conjugated carbonyl compounds have been widely studied as cathodes for AZOBs owing to their good reversibility, diverse structure, and outstanding theoretical capacity
Figure 6. The molecular structure of common carbonyl compounds in AZOBs[30,63-75]. AZOBs: Aqueous zinc-organic batteries; C4Q: calix[4]quinone; 9,10-AQ: 9,10-anthraquinone; 1,2-NQ: 1,2-naphthoquinone; 9,10-PQ: 9,10-phenanthrenequinone; PBQS: poly(benzoquinonyl sulfide); TABQ: tetraamino-p-benzoquinone; 1,4-NQ: 1,4-naphthoquinone; APh-NQ: 2-[(4-hydroxyphenyl) amino] naphthalene-1,4-dione; PTO: pyrene-4,5,9,10-tetraone; NTCDI: 1,4,5,8-naphthalene diimide; PDB: 2,5-dihydroxy-1,4-benzoquinonyl; PDBS: poly(2,5-dihydroxy-1,4-benzoquinonyl sulfide); PT: polythiophene; DTT: dibenzo[b,i]thianthrene-5,7,12,14-tetraone; 4S6Q: 6a,16a-dihydrobenzo[b]naphtho[2′,3′:5,6][1,4]dithiino[2,3-i]thianthrene-5,7,9,14,16,18-hexaone; PQ-Δ: triangular macrocyclic phenanthrenequinone; rPOP: redox-active quinone-based porous organic polymer; PTCDA: perylene-3,4,9,10-tetracarboxylic dianhydride; HqTp: Hq-2,5-diaminohydroquinone, Tp-1,3,5-triformylphloroglucinol; BT-PTO: benzenetricarboxaldehyde pyrene-4,5,9,10-tetraone; COF: covalent-organic frameworks.
Zhao et al. explored for the first time various quinone compounds, including 9,10-anthraquinone
Figure 7. (A) The Zn2+ storage mechanism of the C4Q cathode. Reproduced with permission[30]. Copyright 2018, AAAS; (B) The Zn2+ storage mechanism of the PDBS-Zn. Reproduced with permission[69]. Copyright 2021, Wiley Online Library; (C) Cycling performance of NTCDI cathode. Reproduced with permission[67]. Copyright 2019, Wiley Online Library; (D) Cycling performance of BT-PTO COF cathode under 5 A·g-1. Reproduced with permission[75]. Copyright 2022, Wiley Online Library. C4Q: Calix[4]quinone; PDBS: poly(2,5-dihydroxy-1,4-benzoquinonyl sulfide); NTCDI: 1,4,5,8-naphthalene diimide; BT-PTO: benzenetricarboxaldehyde pyrene-4,5,9,10-tetraone; COF: covalent-organic frameworks.
Compared with quinone compounds, ketone compounds usually exhibit good stability in electrolytes. For instance, PTO cathodes can maintain an ~70% capacity retention after 1,000 cycles under 3 A·g-1, which verifies a decent cycling life[39]. Compared with quinones and ketones, nitrogen atoms in imide compounds have lone pair electrons, which can improve the storage capacity of Zn2+ by enhancing the redox ability of carbonyl groups. Wang et al. prepared 1,4,5,8-naphthalene diimide (NTCDI) as the cathode for AZOBs[67]. The large π-conjugated structure makes NTCDI flexible and guarantees its durability during continuous discharge and charge, maintaining a 73.7% capacity retention under 1 A·g-1 after 2,000 cycles [Figure 7C]. Covalent-organic frameworks (COF) containing carbonyl groups are also popular cathode materials for AZOBs. Zheng et al. synthesized the BT-PTO COF cathode using a benzenetricarboxaldehyde (BT) node and PTO monomer[75]. Thanks to the ordered channel structure that promotes the ion transfer, the cathode provided a prominent cycle lifespan, with a 98.0% capacity retention over 10,000 cycles under 5 A·g-1 [Figure 7D]. However, the synthesis of COFs is usually complicated and tedious, and the inactive node components in these frameworks would increase molecular weight and reduce their theoretical capacity.
Imine compounds
The active functional group (C=N) of imine compounds can obtain electrons and convert to anions (-C-N-) during discharging, accompanied by the insertion of cations (Zn2+ or H+) to maintain charge neutrality. Additionally, the presence of N atoms can improve the electron conductivity and increase the redox potential[20]. In recent years, imines have shown broad applications in AZOBs [Figure 8][32,44,77-87].
Figure 8. The molecular structures of common imine compounds in AZOBs[32,44,77-87]. AZOBs: Aqueous zinc-organic batteries; PNZ: phenazine; DAP: 2,3-diaminophenazine; PoPD: poly(o-phenylenediamine); PTO: pyrene-4,5,9,10-tetraone; HATN: hexaazatrinaphthalene; HATN-3CN: diquinoxalino[2,3-a:2′,3′-c]phenazine-2,8,14-tricarbonitrile; HATTA: 5,6,11,12,17,18-hexaazatrinaphthylene-2,8,14-tricarboxylic acid; HFHATN: hexafluorohexaazatrinaphthylene; HMHATN: hexamethoxy hexaazatrinaphthylene; BBQP: benzo[a]benzo[7,8]quinoxalino[2,3-i]phenazine-5,6,8,14,15,17; HA-COF: hexaazatriphenylene-based covalent-organic frameworks; PA-COF: phenanthroline covalent-organic frameworks.
Small organic 2,3-diaminophenazine (DAP) has been widely used as cathode materials for AZOBs. However, the amino groups in the molecular structure of DAP increase the possibility of hydration by forming hydrogen bonds [Figure 9A], promoting the dissolution in aqueous electrolytes, resulting in poor cycling performance (a 29% capacity retention under 0.1 A·g-1 over 200 cycles)[77]. Liang et al. used a CNTs-modified glass fiber membrane to suppress the dissolution of DAP [Figure 9B] and achieved a decent cycling lifespan (an 80% capacity retention over 10,000 cycles under 25.5 A·g-1)[78]. In addition to the membrane modification, extending the π-conjugated structure of organics is an effective method to suppress dissolution and enhance the electrochemical performance. Tie et al. prepared the HATN with a
Figure 9. (A) Hydration mechanisms of DAP in aqueous electrolytes. Reproduced with permission[77]. Copyright 2020, Elsevier; (B) Schematic diagram of Zn/DAP battery. Reproduced with permission[78]. Copyright 2022, Elsevier; (C) Calculated structural evolution and protonation pathway at discharging process of HATN. Reproduced with permission[32]. Copyright 2020, Wiley Online Library; (D) Calculated molecular electrostatic potential distribution of HATN-3CN molecule. Reproduced with permission[44]. Copyright 2021, Elsevier; (E) Zinc storage mechanism of PoPD. Reproduced with permission[81]. Copyright 2020, Elsevier; (F) (Dis-)charge profiles of
Compounds with dual redox groups
Organic materials with a single redox group (C=O or C=N) have been extensively explored, but their specific capacity is usually limited. Designing organic compounds with multiple redox groups can achieve higher energy density and working voltage. In recent years, some compounds with dual redox groups (C=O and C=N) have recently been developed as cathodes for AZOBs [Figure 10][33,45,86,88-94].
Figure 10. The molecular structures of commonly reported compounds with dual redox groups in AZOBs[33,45,86,88-94]. AZOBs: Aqueous zinc-organic batteries; TAPQ: 5,7,12,14-tetraaza-6,13-pentacenequinone; BPD: benzo[b]phenazine-6,11-dione; PTONQ: benzo[i]benzo
Gao et al. combined the structures of quinone and pyrazine to synthesize a novel organic cathode material for AZOBs, named 5,7,12,14-tetraaza-6,13-pentacenequinone (TAPQ)[33]. TAPQ with abundant C=N and C=O groups provides a significant reversible specific capacity of 443 mAh·g-1. However, the poor reversibility of excessive H+ insertion leads to the collapse of TAPQ cathode structure and unsatisfactory cycling lifespan in 0.1-1.6 V [Figure 11A]. Subsequently, Wang et al. enhanced the cycling lifespan of TAPQ by polymerization[93]. They synthesized poly(triazine-5,7,12,14-tetraaza-6,13-pentacenequinone) (TTPQ) based on TAPQ monomers as cathodes for AZOBs [Figure 11B]. The TTPQ provided a decent cycle stability, with an ~94% capacity retention under 0.5 A·g-1 over 250 cycles. Recently, Li et al. prepared a hydrogen-bonded organic framework, benzo[a]benzo[7,8]quinoxalino[2,3-i]phenazine-5,6,8,14,15,17-hexane (BBQPH), as the cathode for AZOBs[95]. The intermolecular hydrogen bonding enhances the structural stability of BBQPH, and the abundant C=N and C=O groups trigger multi-electron redox chemistry with super delocalization. Therefore, BBQPH cathodes demonstrated a high reversible capacity of 498.6 mAh·g-1 and a high energy density of 355 Wh·kg-1 under 0.2 A·g-1. Similarly, Chen et al. designed HATNQ as cathode material for AZOBs. The two-dimensional layered supramolecular structure of HATNQ is conducive to rapid charge transfer and ion transport [Figure 11C]. Compared with HATN, the HATNQ electrode had a lower polarization and higher operating voltage [Figure 11D][45]. Impressively, the HATNQ cathode displayed a magnificent capacity of 482.5 mAh·g-1 under 0.2 A·g-1 [Figure 11E]. In addition, introducing additional redox groups can increase the capacity of COF cathodes. For example, Wang et al. obtained quinone-functionalized COF (HAQ-COF) by introducing quinone groups to replace the inactive components in hexaazatriphenylene-based COF (HA-COF), significantly improving their electrochemical performance[86]. As presented in Figure 11F, HA-COF and HAQ-COF were prepared through solvent thermal condensation of 1,2,4,5-tetraaminobenzene and 1,2,4,5-tetramino-benzoquinone with hexaketocyclohexane octahydrate, respectively. Compared with HA-COF, HAQ-COF showed better uptake ability for Zn2+ and H+ due to the existence of quinone groups. Therefore, HAQ-COF delivered a superb capacity of 344 mAh·g-1 under 0.1 A·g-1 [Figure 11G], higher than that of HA-COF (195 mAh·g-1, Figure 11H). The preparation of organics with dual redox groups is still complex and costly. In addition, the ion storage mechanisms of dual redox groups are controversial and need further exploration.
Figure 11. (A) Cyclic performance of TAPQ cathode under 0.05 A·g-1. Reproduced with permission[33]. Copyright 2021, Elsevier; (B) Schematic diagram of Zn//TTPQ battery. Reproduced with permission[93]. Copyright 2022, Royal Society of Chemistry; (C) Diagram of two-dimensional layered supramolecular structure of HATNQ. (D) The cyclic voltammetry curves of HATN and HATNQ at 0.2 mV·s-1; (E) (Dis-)charge profiles of the HATNQ cathode at 0.2 A·g-1. Reproduced with permission[45]. Copyright 2022, Wiley Online Library; (F) Synthesis route of HAQ-COF and HA-COF; (Dis-)charge profiles of HAQ-COF (G) and HA-COF (H) cathode under 0.1 A·g-1. Reproduced with permission[86]. Copyright 2021, Wiley Online Library. TAPQ: 5,7,12,14-tetraaza-6,13-pentacenequinone; TTPQ: poly(triazine-5,7,12,14-tetraaza-6,13-pentacenequinone); HATN: diquinoxalino[2,3-a:2’,3’-c]phenazine; HATNQ: hexaazatrinaphthalene-quione; HAQ-COF: quinone-functionalized covalent-organic frameworks; HA-COF: hexaazatriphenylene-based covalent-organic frameworks.
Other n-type compounds
In addition to organics with the most common C=O or C=N groups, several n-type organics with other functional groups (e.g., nitro groups, azo groups, and hydroxyl groups) have also demonstrated the capability of storing Zn2+ and/or H+ ions. Song et al. found that para-dinitrobenzene (p-DB) can be used as redox-active molecules in AZOBs [Figure 12A][34]. They found the nitro groups possessed strong redox activity that enables two consecutive two-electron Zn(OTF)+ (triflate, OTF-=CF3SO3-) reaction mechanisms, which shared Zn2+ with adjacent nitro groups. Impressively, p-DB encapsulated in carbon nanoflower (CF) provided an excellent cycling lifespan under 5 A·g-1 over 25,000 cycles [Figure 12B]. Although many organic cathodes have shown promising performance, their recycling process often faces the problem of complete destruction or decomposition of electrode materials. To this end, Chen et al. proposed a low-cost, recyclable Zn// azobenzene organic battery [Figure 12C][35]. In situ characterizations and quantitative analyses proved that azobenzene can be transformed into hydro-azobenzene by storing protons. Because azobenzene and the discharge product hydro-azobenzene can exist stably in air, and they have very good solubility in organic solvents, the battery can be efficiently recovered under any charge and discharge state, and the average yield of recycled active material in different solvents is as high as 89.1% [Figure 12D]. Interestingly, some originally inert groups can become active centers after proper electrochemical activation. For example, Sun et al. reported a hydroxyl polymer polytetrafluorohydroquinone (PTFHQ) as a cathode for AZOBs[96]. The part of hydroxyl groups in PTFHQ can be oxidized to active carbonyl groups through an in-situ activation process; thereby, Zn2+ can be stored/released. Additionally, the Z-fold structure of PTFHQ is essential for rapid ion diffusion [Figure 12E]. As a result, the PTFHQ cathode offered an outstanding rate capacity (196 mAh·g-1 under 20 A·g-1, Figure 12F). This work expands the understanding of the working mechanism of AZOBs and offers a constructive method for applying polymer cathode materials.
Figure 12. (A) Proposed redox reaction of p-DB; (B) Cycling performance of p-DB@CF cathode under 5 A·g-1. Reproduced with permission[34]. Copyright 2022, Wiley Online Library; (C) Diagram of a recyclable and scalable Zn//AZOB battery; (D) Recovery rate of active substances in different solvents. Reproduced with permission[35]. Copyright 2023, Wiley Online Library; (E) The synthesis of PTFHQ and proposed redox mechanism; (F) Rate capability of PTFHQ cathode. Reproduced with permission[96]. Copyright 2023, Wiley Online Library. p-DB: Para-dinitrobenzene; AZOB: aqueous zinc-organic battery; PTFHQ: hydroxyl polymer polytetrafluorohydroquinone.
As discussed above, n-type organics exhibit high specific capacity and outstanding rate capacity. However, similar to p-type organics, some n-type organics and their discharge products dissolve severely in aqueous electrolytes, resulting in poor cycle life. This can be improved through structural optimization (such as co-polymerization and expanding π-conjugated structures of molecules) and separator design[45,69,78]. Hybridizing organics with carbons can also enhance the stability and improve conductivity[65]. Additionally, the low working voltage of n-type organics results in a lower energy density of the battery. Introducing electron-withdrawing conjugated groups can increase the working voltage, but its improvement is generally limited[32,44]. Organics integrating carbonyl and imine compounds exhibit relatively high voltage and capacity, with high energy density, which can be further explored in future work.
Bipolar-type organic cathodes
Bipolar organic molecules contain n-type and p-type redox components that can serve as either electron acceptors or electron donors. Bipolar organics combine the advantages of p-type organics and n-type organics and, therefore, often show improved capacity and operating voltage [Figure 13][97-103].
At present, various bipolar polymers [e.g., polyaniline (PANI)[97], poly(p-phenylene) (PPP)[98], etc.] are reported as cathodes for AZOBs, among which PANI is the first reported one. The PANI-Zn battery in earlier research was operated in ZnCl2/NH4Cl electrolyte[27]. In order to evidently explain the electrochemical mechanism, Wan et al. designed and synthesized PANI/carbon felt (PANI/CF) electrodes and explored the mechanism by ex situ spectroscopy[97]. During the initial discharging process, Cl- was removed from PANI, and Zn2+ may interact with PANI. During the charging process, Zn2+ was removed from PANI and CF3SO3- was embedded [Figure 14A]. This dual-ion insertion/extraction mechanism is conducive to fast transfer of charge carriers. Therefore, PANI/CFs exhibited decent rate ability (95 mAh·g-1 under 5 A·g-1). Noteworthy, the mass loading of most reported AZOB organic cathodes is relatively low (about 2 mg·cm-2), far away from the requirements for commercial use (10 mg·cm-2, as referred to LIBs). Yan et al. reported a polymer poly(1,8-diaminonaphthalene) (PDAN) as a cathode for AZOBs, showing a reaction mechanism similar to that of PANI [Figure 14B][103]. Benefiting from the bipolar-type reaction mechanism and semi-conductive property of PDAN, Zn//PDAN batteries with high-quality load
Figure 14. (A) Redox mechanism of PANI/CFs. Reproduced with permission[97]. Copyright 2018, Wiley Online Library; (B) Redox mechanism of PDAN. Reproduced with permission[103]. Copyright 2022, Wiley Online Library; (C) Bipolar ion storage mechanism of Thn-CH3COO. Reproduced with permission[102]. Copyright 2023, Wiley Online Library. PANI/CFs: Polyaniline/carbon nanoflower; PDAN: polymer poly(1,8-diaminonaphthalene).
In general, the redox process of bipolar-type organic cathodes involves the insertion/extraction of cations and anions. They often show superior performance than n- or p-type organic cathodes. It should be noted that most bipolar-type organics are polymers that require many inactive components to stabilize the structures, which compromises the specific capacity. In addition, the synthesis of bipolar-type organics is generally much more complex, and thus, they have been considerably less explored as cathodes for AZOBs. In future research, it may be considered to maximize the specific capacity of bipolar-type organic cathodes while ensuring high voltage and conductivity, such as developing bipolar-type organics with low molecular weight and solubility.
The characteristics of three types of organic cathodes are shown in Figure 15 (also see a detailed comparison of the electrochemical performance of various organic cathodes in Table 1). To summarize, p-type organic cathodes necessitate a substantial amount of salt from the electrolyte during the charging process, which escalates the assembly cost of the battery[38]. Despite demonstrating high voltages and conductivity, their limited active center results in unsatisfactory capacity and low energy density. However, their high electron affinity and excellent biocompatibility make them promising candidates for wearable medical devices. In contrast, n-type organic cathodes have a relatively straightforward preparation process and typically offer higher capacity, energy density, and longer cycling lifespan[45,95], albeit at a lower operating voltage. Given these characteristics, n-type organic cathodes are well-suited for energy storage systems that demand high energy density and long cycle life, such as those used in residential and commercial settings. Furthermore, bipolar-type organic cathodes amalgamate the advantages of both n- and p-type organic cathodes, exhibiting high operating voltage, high conductivity, and robust cycle life. They are ideal for energy storage systems that require high electrical conductivity and stability, such as medical devices and sensor equipment.
Electrochemical performance of organic cathodes for AZOBs
Cathode | Type | Electrolyte | Capacity (mAh·g-1/A·g-1) | Rate capacity (mAh·g-1/A·g-1) | Cycling stability (%/no. cycle/A·g-1) | Ref. |
PTVE | p | 1M Zn(CF3SO3)2 | 83/0.2 | 52/10 | 77.0/1,000/1 | [47] |
Thianthrene | 1M Zn(OTF)2-acetonitrile | 112/0.5 | 66/20 | 82/8,000/1 | [51] | |
BDB | 1M Zn(OTF)2-19M LiN(SO3CF3) | 125/0.026 | 80/0.78 | 75/1,000/0.78 | [31] | |
m-PTPA | 2M ZnCl2 | 210.7/0.5 | 107.5/6 | 87.6/1,000/6 | [52] | |
DMPZ | 17M NaClO4 | 231/0.051 | 107/1.275 | 81/1,000/0.255 | [53] | |
PPy | - | 123/1.9 | 40/44.7 | 38/200/4.4 | [55] | |
Poly(5-cyanoindole) | 1M ZnCl2 | 107/0.2C | 61/10C | 63/800/2C | [58] | |
C4Q | n | 3M Zn(CF3SO3)2 | 335/0.02 | 172/1 | 87/1,000/0.5 | [30] |
NQ@CNT | 2M ZnSO4 | 333.5/0.34 | 129.7/0.68 | 41/1,500/0.339 | [65] | |
PDBS | 2M ZnSO4 | 260/0.01 | 161.25/5 | 79/2,000/2 | [69] | |
PTO | 2 M ZnSO4 | 336/0.04 | 113/20 | 70/1,000/3 | [39] | |
NTCDI | 2 M ZnSO4 | 240/0.1 | 140/2 | 73.7/2,000/1 | [67] | |
BT-PTO COF | 3 M Zn(CF3SO3)2 | 225/0.1 | 108/500 | 98/10,000/5 | [75] | |
DAP | 3 M Zn(CF3SO3)2 | 219.1/0.255 | 77/127.5 | 80/10,000/25.5 | [78] | |
HATN | 2 M ZnSO4 | 405/0.1 | 123/20 | 93.3/5,000/5 | [32] | |
HATN-3CN | 2 M ZnSO4 | 320/0.05 | 190/20 | 90.7/5,800/5 | [44] | |
HATTA | 1M Zn(CF3SO3)2 | 225.8/0.05 | 136.1/25 | 84.07/10,000/25 | [79] | |
HATN-PNZ | 2 M ZnSO4 | 226/5 | 159/30 | 92.7/30,000/30 | [80] | |
PoPD | 2 M ZnSO4 | 318/0.05 | 95/5 | 66.2/3,000/1 | [81] | |
PA-COF | 1 M ZnSO4 | 247/0.1 | 68/10 | 62/10,000/1 | [87] | |
TAPQ | 1 M ZnSO4 | 443/0.05 | 15/5 | 50.2/100/0.05 | [33] | |
TTPQ | 2 M ZnSO4 | 404/0.3 | 204/5 | 94/250/0.5 | [93] | |
HATNQ | 3 M ZnSO4 | 482.5/0.2 | 177.5/9 | 80/11,000/5 | [45] | |
HA-COF | 2 M ZnSO4 | 164/0.1 | 35.4/10 | 75.3/10,000/5 | [86] | |
HAQ-COF | 2 M ZnSO4 | 344/0.1 | 95.6/10 | 85/10,000/5 | [86] | |
p-DB@CF | 3 M Zn(CF3SO3)2 | 402/0.1 | 214/20 | 93.9/25,000/5 | [34] | |
PTFHQ | 2 M Zn(CF3SO3)2 | 215/0.1 | 145/50 | 92/3,400/20 | [96] | |
PANI/CFs | bi | 1 M Zn(CF3SO3)2 | 200/0.05 | 95/5 | 92/3,000/5 | [97] |
PDAN | 0.5 M Zn(CF3SO3)2 | 171/0.1 | 54/100 | 82.3/10,000/5 | [103] | |
Thn-CH3COO | 2 M Zn(CF3SO3)2 | 162/0.1 | 113.4/5 | 65/500/1 | [102] |
IMPROVEMENT STRATEGIES FOR HIGH PERFORMANCE
Although organic cathodes have shown significant advantages for AZOB application, some issues remain unsolved, such as the dissolution of small molecular compounds, low operating voltage, poor conductivity, etc., which lead to poor cycling lifespan and low energy density of the AZOBs. This chapter will discuss strategies to improve the voltage, specific capacity, cycling lifespan and rate capability of organic cathodes [Figure 16], which are crucial parameters for evaluating battery performance.
Figure 16. Strategies for improving the voltage, specific capacity, cycling lifespan and rate capability.
Voltage
The cathode voltage (Ecathode) is determined by the Gibbs free energy change (ΔG) during the electrochemical reactions, as formulated in
where F and n represent the Faraday constant and the number of transferred electrons, respectively[104]. Therefore, high-voltage organic cathodes can be identified by screening their corresponding ΔG/n values. Cui et al. explored the effect of electron cloud distributions on the voltage of organic sulfur compounds (thianthrene, phenoxathiine and phenothiazine)[51]. The electronegativity of thianthrene (-13.30) with a symmetrically distributed electron cloud distribution is greater than that of phenoxathiine (-16.72) and phenothiazine (-20.21). Due to the difference in electronegativity, the ΔG of thianthrene is greater than that of phenoxathiine and phenothiazine, and the discharge voltage of thianthrene (1.5 V) is notably higher than that of phenoxathiine (1.3 V) and phenothiazine (0.85 V). Optimization of electrolyte composition can also increase ΔG/n, which is an efficacious method to enhance the working voltage. For example, DFT calculation results show that ΔG/n of [2(PTVE+)SO42-] is higher than that of (PTVE+ClO4-) and
Specific capacity
The theoretical capacity (QTheory) of organic cathodes is obtained as
where n, F, and M represent the theoretically maximal number of electrons transferred during charging/discharging, Faraday constant, and relative molecular mass, respectively. Therefore, increasing the “n” and decreasing the “M” of organics are conducive to obtaining high theoretical specific capacity. Increasing the redox-active sites of organics can increase the “n” value, thereby increasing the theoretical specific capacity. For example, PTO, obtained by introducing two carbonyl groups in 9,10-PQ, has a theoretical capacity of 409 mAh·g-1[39], higher than 9,10-PQ (258 mAh·g-1)[30]. Similarly, compared to HATN (419 mAh·g-1), HATNQ has six more carbonyl groups and a higher theoretical capacity of 515 mAh·g-1[32,45]. Removing inactive components from organics can reduce the “M”; it is also a method to enhance theoretical specific capacity. For example, PNZ can be obtained by removing the inactive amino components in DAP molecules, and the theoretical specific capacity is increased from 255 to 297 mAh·g-1[77,78]. However, dissociating some small molecular organics in aqueous electrolytes usually leads to poor cycle lifespan. Cycling stability needs to be considered when the “M” is reduced.
The above two approaches can enhance the theoretical specific capacity of organics. However, the actual specific capacity is influenced by many factors, such as electrolytes, conductivity, and morphology. Combining organics with highly conductive carbon materials is the most direct method to improve the conductivity. For instance, the initial capacity of NQ@CNT composite cathodes is 333.5 mAh·g-1, close to their theoretical capacity (339.0 mAh·g-1)[65]. Similarly, the actual specific capacity of the PANI/graphene composite cathode (184.5 mAh·g-1) is also higher than that of the PANI cathode (163.1 mAh·g-1)[107]. Besides, the morphology of organics also affects their actual specific capacity. For instance, the specific capacity of “pebble-like” PANI is half that of “sponge-like” PANI because electrolytes and solvents can more easily enter the porous structure[108]. Moreover, the m-PTPA conjugated microporous polymer (CMP) cathode with a conjugated microporous structure exhibits a discharge capacity of 210.7 mAh·g-1 at 0.5 A·g-1, which is higher than the nonporous conjugated PTPA cathode (99.8 mAh·g-1)[52]. In addition, Zhang et al. synthesized cuboid, sphere, and narrow strip-shaped PoPD (PoPDH, PoPDN and PoPDA) in acidic, neutral, and alkaline solvents as cathodes for AZOBs, respectively[81]. The cuboid PoPDH cathode, due to its higher conductivity compared to the spherical PoPDN and narrow strip PoPDA cathodes, has a higher discharge capacity at 0.05 A·g-1. The capacities are as follows: cuboid PoPDH (318 mAh·g-1), spherical PoPDN
Cycling lifespan
The cycle lifespan of AZOBs is significant for their practical application, which is determined by their components, including the organic cathodes, Zn anodes, electrolytes, and separators. Some of the organic materials suffer from dissolution issues and, consequently, unsatisfactory cycling lifespan in liquid electrolytes, mainly because of the intrinsic instability of the molecular structure due to the weak intermolecular van der Walls forces. In addition, they may also be detached from the electrode by the solvation of solvent molecules in electrolytes[111]. Molecular polymerization can expand the molecular size of monomers and hinder the solvation process, thereby suppressing the dissolution and enhancing structural stability. For example, the serious dissolution of 2,5-dichloro-3,6-dihydroxy-p-quinone (CLA) monomers in aqueous electrolytes leads to rapid capacity decline. To solve this problem, Sun et al. prepared PDBS based on CLA monomers as the cathode for AZOBs[69]. Compared with CLA, the stability of PDBS cathodes was significantly enhanced (a 79% capacity retention under 2 A·g-1 after 2,000 cycles). Additionally, COFs connected by covalent bonds to form a spatial network structure reveal good stability. For example, compared to PTO, BT-PTO COF has a remarkable cycle lifespan, with a 98% capacity retention under
As a part of the AZOBs structure, separators also significantly influence the cycle lifespan. Through delicate separator design, the dissolution of active substances and the shuttle of dissolved substances to the counter-electrode could be effectively suppressed. Liang et al. used a modified glass fiber membrane with CNTs film to inhibit the dissolution of DAP, providing an outstanding cycling lifespan (an 80% capacity retention under 25.5 A·g-1 over 10,000 cycles)[78]. With a cation-selective membrane (Nafion membrane), Zn//C4Q batteries can provide a cycling lifespan of 1,000 cycles under 0.5 A·g-1 (87% capacity retention)[30]. Similarly, Zn//copper-tetracyanoquinodimethane (CuTCNQ) batteries using a graphene-modified glass fiber membrane showed high rate performance and decent cycling lifespan, as the modified membrane can inhibit the dissolution of CuTCNQ and the shuttle effect in aqueous electrolytes[112]. However, ion-selective membranes and graphene-modified membranes cannot fundamentally solve the dissolution problem, and their cost is generally much higher than that of commonly used glass fiber membranes. In addition, different types and concentrations of electrolytes can affect the cycling lifespan of AZOBs. For example, the cycling lifespan of the PANI/CFs in Zn(CF3SO3)2 electrolytes is better than that in ZnSO4 electrolytes because the CF3SO3- can stabilize the oxidized PANI by reducing the number of water molecules around oxidized PANI[97]. The number of free solvent molecules in high-concentration electrolytes greatly decreases with changes in solvation structure, which can alleviate the dissolution of organics. For example, 3,4,9,10-perylenetetracarboxylic dianhydride exhibited excellent cycling lifespan in high concentration electrolytes, with an 87% capacity retention under 1 A·g-1 over 1,000 cycles[113]. In addition, electrolytes can also be optimized from the perspectives of ionic liquids[114-116], electrolyte additives[117-119], organic solvents[120-122], and quasi/all-solid electrolytes[123-125]. Additionally, 5,7,12,14-pentacenetetrone cathodes were found to be more stable in 1 M Zn(CF3SO3)2 electrolytes containing trimethyl phosphate (TMP) additives (with a 61.86% capacity retention under 0.1 A·g-1 after 550 cycles) than in 1 M Zn(CF3SO3)2 electrolytes[126], due to the strong interaction between Zn2+ and TMP that changes the solvation structure and inhibits parasitic reactions. As an important component of batteries, Zn anodes also restrict the cycling lifespan of AZOBs to a certain extent. During the cycling process, Zn anodes typically face issues such as hydrogen evolution reaction, zinc dendrites growth, corrosion, and byproduct formation, leading to a decrease in cycling lifespan[127-131]. At present, the protection of Zn anodes is mainly achieved through strategies such as artificial surface coatings, controllable synthesis, advanced separators, and electrolyte engineering[132-136]. For instance, a Na3Zr2Si2PO12 (NZSP) coating was shown to effectively suppress the hydrogen evolution and the formation of zinc dendrites, resulting in a prolonged lifespan of NZSP-Zn//V2O5@PEDOT batteries compared to Zn//V2O5@PEDOT[136]. Overall, in addition to designing structurally stable organic cathode materials, separator modification, electrolyte optimization, and Zn anode protection are also promising strategies for improving the long cycle lifespan of AZOBs.
Rate capability
The rate capability is a key indicator for evaluating the fast-charging capacity of batteries, mainly related to the mobility of ions and electrons. In general, the electronic conductivity of most organic compounds is relatively poor, and their rate capability is often unsatisfactory. Expanding the π-conjugated structures in organics is an efficacious way to enhance its intrinsic conductivity, which can increase π-electron delocalization and thus improve electron migration and further the conductivity of organics[44,137]. For example, HATTA cathodes obtained by introducing electron-withdrawing conjugative groups (-COOH) into the HATN molecule delivered a capacity of 136.1 mAh·g-1 under 25 A·g-1, higher than HATN cathodes (123 mAh·g-1 under 20 A·g-1)[79]. Besides, HATN-PNZ with large charge delocalization area can effectively transfer electrons in molecules due to the conjugation elongation of aromatic rings and phenazine, leading to rapid kinetic reactions. Compared with PNZ (97 mAh·g-1 under 100 A·g-1), HATN-PNZ offered a superior rate capacity of 144 mAh·g-1 under 100 A·g-1[80].
Optimizing synthesis methods and conditions to control the crystal structure and morphology of organic materials is also a viable strategy to enhance conductivity. For instance, Luo et al. prepared organic cathodes of perylene-3,4,9,10-tetracarboxylic dianhydride (PTCDA) with varying degrees of crystallinity. Compared to pure PTCDA, the variant with higher crystallinity expands the π-π conjugated system, leading to more delocalized electrons. This facilitates the transfer of electrons and ions, thereby improving the conductivity and providing superior rate capability[138]. Given that protons can serve as charge carriers, the conductivity of PoPD synthesized under acidic conditions significantly surpasses that of its counterpart synthesized under neutral/alkaline conditions. This results in a high capacity of 318 mAh·g-1 at 0.05 A·g-1 and decent rate performance (95 mAh·g-1 at 5 A·g-1)[81]. Doping organic polymers with metal ions or organic molecules can alter the structure and carrier concentration of the organics, thereby enhancing their conductivity. For example, Gong et al. designed a Zn2+-doped polyaniline (PAZ) organic cathodes using a re-doping method, which resulted in high conductivity. Consequently, with the current density increasing from 1.0 to 10 A·g-1, the Zn//PAZ battery can deliver a capacity of 285.4 to 134.1 mAh·g-1[139]. Similarly, Ma et al. leveraged the semi-self-doping properties of phytic acid to effectively boost the conductivity of PANI cathode by doping it with phytic acid. This resulted in a superior rate capacity, with a specific capacity of ~150/114 mAh·g-1 at
The most used strategy for improving conductivity is to composite organics with conductive carbon materials. These materials typically have superior conductivity and large specific surface area, allowing electrons to quickly transfer along the network, thereby improving rate capability. For example, the poly quinone phenylenediamine (PONEA)/graphene composite cathodes can exhibit a splendid capacity of
CONCLUSIONS AND PERSPECTIVES
In recent years, organic cathode materials have shone in the field of aqueous zinc ion batteries because of their advantages of environmental friendliness, wide sources, adjustable structure, and high capacity. In this review, we first introduced the development history of AZOBs and then summarized the recent progress in three typical types of organic cathodes, namely the p-, n-, and bipolar-type organics, in terms of both the working mechanisms and electrochemical performance. These organic materials have their pros and cons. For instance, n-type organic materials have drawn increasing attention due to their high capacity, fast rate capability, environmental friendliness, and abundance. However, they still suffer from low electrical conductivity and high solubility in electrolytes. P-type organic materials, on the other hand, offer different advantages and challenges. While they can also exhibit high redox voltage and fast rate capability, they may face issues related to stability and reversibility of the redox reactions. Bipolar-type organic materials can potentially combine the advantages of both p-type and n-type materials, offering a balance between performance, stability, and cost. However, the design and synthesis of bipolar-type materials can be more complex. In this regard, we further discussed the developed strategies for improving the performance of AZOBs based on the voltage, specific capacity, cycling lifespan and rate capability.
Though the research of organic cathodes in AZOBs has made great progress, the commercial application of AZOBs still faces many challenges: (1) The energy density is relatively low. Due to using aqueous electrolytes, the working voltage of the AZOBs is normally less than 2.0 V. Moreover, additional conducting additives are often required to enhance the conductivity of organic cathodes, which, however, might reduce the energy density; (2) Working mechanism is not fully understood. Currently, it is proposed that these materials may store Zn2+ and/or H+ ions. However, it remains unclear which mechanism dominates. Furthermore, the regulation of the reaction mechanism is also not well understood; (3) Unexpected side effects. During the charging and discharging process, some small organics and discharge products are often dissolved in aqueous electrolytes, resulting in a poor cycling lifespan.
In response to the above challenges, we propose the following prospects for the future development of organic cathodes.
(1) Improving the energy density of organic cathodes. Many organic materials suffer from low conductivity and thus require a lot of conductive carbon during the electrode fabrication process, which, in turn, would reduce the active materials and, consequently, the energy density of the battery. Several strategies could be considered to address this issue.
(a) Advanced manufacturing techniques. Techniques such as nanostructuring or creating composite materials could be used to improve the distribution of conductive carbon in the electrode, which could enhance conductivity without significantly reducing the proportion of active materials.
(b) Surface modification. Surface modification of active materials could be another way to improve their conductivity. This could involve coating the surface of the active materials with a thin layer of conductive material or altering the surface chemistry to improve its interaction with conductive carbon.
(c) Structural optimization. The structure of the active materials could be optimized to improve their interaction with conductive carbon, thereby enhancing the overall conductivity of the electrode without needing to add excessive amounts of conductive carbon.
(d) Developing new materials: research could focus on developing new organic materials with higher intrinsic conductivity, which would reduce the need for additional conductive carbon.
These are just a few potential strategies, and research in this area is ongoing. The goal is to find a balance that allows for high conductivity while maintaining a high proportion of active materials, thereby maximizing the energy density of the battery. It is also worth mentioning that machine learning could be a very promising approach to facilitating the discovery of new and high-performance organic cathodes. By analyzing the electrochemical data of known organic materials and furthering the structure-property relationship, key elements that affect the battery performance can then be identified. Consequently, new organic structures with these key elements can be predicted and further experimentally verified. It is noted that the application of machine learning in new organics design is still in its early stages, requiring high-quality and diverse data and algorithm support.
(2) Understanding the charge storage and capacity decay mechanisms of organic cathodes. Previous studies reveal that both Zn2+ and H+ storages contribute to the capacity of organic cathodes of AZOBs. The diffusion of protons is kinetically smoother and is beneficial to high-rate performance, but it also induces local pH changes and promotes the generation of byproducts. Therefore, understanding the reaction mechanism and furthering its regulation are essential to achieving high-performance AZOBs. It should be noted that organic cathodes containing carbon elements are often quite sensitive and easy to decompose under certain characterization conditions, such as the high voltage electron beams. This brings about additional difficulty in tracking the dynamic structural evolution of the material during charging and discharging. Some advanced characterization techniques (e.g., in situ Fourier transform infrared spectroscopy, nuclear magnetic resonance, and electrochemical quartz crystal microbalance), together with theoretical simulations, provide approaches to comprehending the detailed energy storage mechanism of organic cathodes and, consequently, the performance optimization.
(3) Enhancing the stability of organic cathodes. The stability and lifespan of organic cathodes in aqueous zinc batteries can be compromised due to the dissolution of some organic materials in aqueous electrolytes. This dissolution is closely tied to the structure and composition of the organic materials. As such, optimizing these aspects could potentially decrease their solubility in the electrolyte, thereby enhancing the cycling lifespan of batteries. Additionally, applying protective layers or coatings on the cathode material can prevent the organic materials from directly contacting the electrolyte, further improving the stability of the organic cathodes. Modifying the electrolyte formula or incorporating specific electrolyte additives may also help minimize the dissolution of the organic materials. However, the key to devising effective mitigation strategies lies in a thorough understanding of the dissolution process.
(4) Exploring other avenues for organic cathodes. Organic cathodes have good flexibility and elasticity, and flexible batteries based on organic cathodes have broader application prospects in wearable devices. Based on their good biocompatibility, organic cathodes can be used in medical devices such as pacemakers to provide continuous electrical energy. In addition, the degradability of organic cathodes is also conducive to the construction of biodegradable batteries, making them easier to handle and recover after the end of battery life, thereby reducing the impact on the environment.
In summary, there is still a long way to go in developing sustainable, economical, and high-performance organic cathodes for AZOBs. We aspire that this review will offer valuable inspiration for the mechanism research and construction of high-performance AZOBs.
DECLARATIONS
Authors’ contributions
Proposed the topic of this review: Liang H
Prepared the manuscript: Li J
Collectively discussed and revised the manuscript: Li J, Liang H
Availability of data and materials
Not applicable.
Financial support and sponsorship
This work was supported by the National Natural Science Foundation of China (Grant No. 22001081) and the Science and Technology Projects of Innovation Laboratory for Sciences and Technologies of Energy Materials of Fujian Province (IKKEM, Grant No. HRTP-[2022]-7).
Conflicts of interest
Both authors declared that there are no conflicts of interest.
Ethical approval and consent to participate
Not applicable.
Consent for publication
Not applicable.
Copyright
© The Author(s) 2024.
REFERENCES
3. Kang S, Cheng J, Gao W, Cui L. Toward safer lithium metal batteries: a review. Energy Mater 2023;3:300043.
4. Feng Y, Zhou L, Ma H, et al. Challenges and advances in wide-temperature rechargeable lithium batteries. Energy Environ Sci 2022;15:1711-59.
5. Xiao D, Lv X, Fan J, Li Q, Chen Z. Zn-based batteries for energy storage. Energy Mater 2023;3:300007.
6. Blanc LE, Kundu D, Nazar LF. Scientific challenges for the implementation of Zn-ion batteries. Joule 2020;4:771-99.
7. Gourley SW, Brown R, Adams BD, Higgins D. Zinc-ion batteries for stationary energy storage. Joule 2023;7:1415-36.
8. Chen L, An Q, Mai L. Recent advances and prospects of cathode materials for rechargeable aqueous zinc-ion batteries. Adv Mater Inter 2019;6:1900387.
9. Han M, Chen D, Lu Q, Fang G. Aqueous rechargeable Zn-iodine batteries: issues, strategies and perspectives. Small 2023:e2310293.
10. Alfaruqi MH, Gim J, Kim S, et al. Enhanced reversible divalent zinc storage in a structurally stable α-MnO2 nanorod electrode. J Power Sources 2015;288:320-7.
11. Cui Y, Ding Y, Guo L, et al. Ultra-long Zn3V2O7(OH)2·2H2O nanowires grown on carbon cloth as cathode material for aqueous zinc-ion batteries. Energy Mater 2023;3:300023.
12. Zhang K, Kuang Q, Wu J, et al. Layered structural Zn2Mo3O8 as electrode material for aqueous zinc-ion batteries. Electrochim Acta 2022;403:139629.
13. Lu Y, Wang J, Zeng S, et al. An ultrathin defect-rich Co3O4 nanosheet cathode for high-energy and durable aqueous zinc ion batteries. J Mater Chem A 2019;7:21678-83.
14. Zhang Y, Wang Y, Lu L, Sun C, Yu DY. Vanadium hexacyanoferrate with two redox active sites as cathode material for aqueous
15. Li Y, Zhao J, Hu Q, et al. Prussian blue analogs cathodes for aqueous zinc ion batteries. Mater Today Energy 2022;29:101095.
16. Xu Y, Huang W, Liu J, et al. Promoting the reversibility of electrolytic MnO2-Zn battery with high areal capacity by VOSO4 mediator. Energy Mater 2024;4:400005.
17. Mathew V, Sambandam B, Kim S, et al. Manganese and vanadium oxide cathodes for aqueous rechargeable zinc-ion batteries: a focused view on performance, mechanism, and developments. ACS Energy Lett 2020;5:2376-400.
18. Schon TB, McAllister BT, Li PF, Seferos DS. Correction: the rise of organic electrode materials for energy storage. Chem Soc Rev 2016;45:6405-6.
19. Li Z, Tan J, Wang Y, et al. Building better aqueous Zn-organic batteries. Energy Environ Sci 2023;16:2398-431.
20. Wang H, Wu Q, Cheng L, Zhu G. The emerging aqueous zinc-organic battery. Coord Chem Rev 2022;472:214772.
21. Tie Z, Niu Z. Design strategies for high-performance aqueous Zn/organic batteries. Angew Chem Int Ed Engl 2020;59:21293-303.
22. Cui H, Ma L, Huang Z, Chen Z, Zhi C. Organic materials-based cathode for zinc ion battery. SmartMat 2022;3:565-81.
23. Zheng S, Wang Q, Hou Y, Li L, Tao Z. Recent progress and strategies toward high performance zinc-organic batteries. J Energy Chem 2021;63:87-112.
24. Cui J, Guo Z, Yi J, et al. Organic cathode materials for rechargeable zinc batteries: mechanisms, challenges, and perspectives. ChemSusChem 2020;13:2160-85.
25. Sun T, Fan HJ. Understanding cathode materials in aqueous zinc-organic batteries. Curr Opin Electrochem 2021;30:100799.
26. Zhang M, Zhang Y, Huang W, Zhang Q. Recent progress in calix[n]quinone (n = 4, 6) and pillar[5]quinone electrodes for secondary rechargeable batteries. Batter Supercaps 2020;3:476-87.
27. Macdiarmid AG, Chiang J, Halpern M, et al. “Polyaniline”: interconversion of metallic and insulating forms. Mol Cryst Liq Cryst 1985;121:173-80.
28. Koshika K, Sano N, Oyaizu K, Nishide H. An ultrafast chargeable polymer electrode based on the combination of nitroxide radical and aqueous electrolyte. Chem Commun 2009:836-8.
29. Häupler B, Rössel C, Schwenke AM, et al. Aqueous zinc-organic polymer battery with a high rate performance and long lifetime. NPG Asia Mater 2016;8:e283.
30. Zhao Q, Huang W, Luo Z, et al. High-capacity aqueous zinc batteries using sustainable quinone electrodes. Sci Adv 2018;4:eaao1761.
31. Glatz H, Lizundia E, Pacifico F, Kundu D. An organic cathode based dual-ion aqueous zinc battery enabled by a cellulose membrane. ACS Appl Energy Mater 2019;2:1288-94.
32. Tie Z, Liu L, Deng S, Zhao D, Niu Z. Proton insertion chemistry of a zinc-organic battery. Angew Chem Int Ed Engl 2020;59:4920-4.
33. Gao Y, Li G, Wang F, et al. A high-performance aqueous rechargeable zinc battery based on organic cathode integrating quinone and pyrazine. Energy Storage Mater 2021;40:31-40.
34. Song Z, Miao L, Duan H, et al. Anionic co-insertion charge storage in dinitrobenzene cathodes for high-performance aqueous zinc-organic batteries. Angew Chem Int Ed Engl 2022;61:e202208821.
35. Chen Y, Dai H, Fan K, et al. Frontispiece: a recyclable and scalable high-capacity organic battery. Angew Chem Int Ed Engl 2023;62:e202302539.
36. Poizot P, Gaubicher J, Renault S, Dubois L, Liang Y, Yao Y. Opportunities and challenges for organic electrodes in electrochemical energy storage. Chem Rev 2020;120:6490-557.
37. Esser B, Dolhem F, Becuwe M, Poizot P, Vlad A, Brandell D. A perspective on organic electrode materials and technologies for next generation batteries. J Power Sources 2021;482:228814.
38. Lu Y, Chen J. Prospects of organic electrode materials for practical lithium batteries. Nat Rev Chem 2020;4:127-42.
39. Guo Z, Ma Y, Dong X, Huang J, Wang Y, Xia Y. An environmentally friendly and flexible aqueous zinc battery using an organic cathode. Angew Chem Int Ed Engl 2018;57:11737-41.
40. Nam KW, Kim H, Beldjoudi Y, Kwon TW, Kim DJ, Stoddart JF. Redox-active phenanthrenequinone triangles in aqueous rechargeable zinc batteries. J Am Chem Soc 2020;142:2541-8.
41. Shi M, Das P, Wu ZS, Liu TG, Zhang X. Aqueous organic batteries using the proton as a charge carrier. Adv Mater 2023;35:e2302199.
42. Deng X, Sarpong JK, Zhang G, et al. Proton storage chemistry in aqueous zinc-organic batteries: a review. InfoMat 2023;5:e12382.
43. Wang Y, Wang C, Ni Z, et al. Binding zinc ions by carboxyl groups from adjacent molecules toward long-life aqueous zinc-organic batteries. Adv Mater 2020;32:e2000338.
44. Ye Z, Xie S, Cao Z, et al. High-rate aqueous zinc-organic battery achieved by lowering HOMO/LUMO of organic cathode. Energy Storage Mater 2021;37:378-86.
45. Chen Y, Li J, Zhu Q, et al. Two-dimensional organic supramolecule via hydrogen bonding and π-π stacking for ultrahigh capacity and long-life aqueous zinc-organic batteries. Angew Chem Int Ed Engl 2022;61:e202116289.
46. Koshika K, Sano N, Oyaizu K, Nishide H. An aqueous, electrolyte-type, rechargeable device utilizing a hydrophilic radical polymer-cathode. Macromol Chem Phys 2009;210:1989-95.
47. Luo Y, Zheng F, Liu L, et al. A high-power aqueous zinc-organic radical battery with tunable operating voltage triggered by selected anions. ChemSusChem 2020;13:2239-44.
48. Winsberg J, Janoschka T, Morgenstern S, et al. Poly(TEMPO)/zinc hybrid-flow battery: a novel, “green,” high voltage, and safe energy storage system. Adv Mater 2016;28:2238-43.
49. C´iric´-Marjanovic´ G, Mentus S. Charge-discharge characteristics of polythiopheneas a cathode active material in a rechargeable battery. J Appl Electrochem 1998;28:103-6.
50. Simons TJ, Salsamendi M, Howlett PC, Forsyth M, Macfarlane DR, Pozo-gonzalo C. Rechargeable Zn/PEDOT battery with an imidazolium-based ionic liquid as the electrolyte. ChemElectroChem 2015;2:2071-8.
51. Cui H, Wang T, Huang Z, et al. High-voltage organic cathodes for zinc-ion batteries through electron cloud and solvation structure regulation. Angew Chem Int Ed Engl 2022;61:e202203453.
52. Zhang H, Zhong L, Xie J, Yang F, Liu X, Lu X. A COF-like N-rich conjugated microporous polytriphenylamine cathode with pseudocapacitive anion storage behavior for high-energy aqueous zinc dual-ion batteries. Adv Mater 2021;33:e2101857.
53. Lee MH, Kwon G, Lim H, et al. High-energy and long-lasting organic electrode for a rechargeable aqueous battery. ACS Energy Lett 2022;7:3637-45.
54. Wang S, Huang S, Yao M, Zhang Y, Niu Z. Engineering active sites of polyaniline for AlCl2+ storage in an aluminum-ion battery. Angew Chem Int Ed Engl 2020;59:11800-7.
55. Wang J, Liu J, Hu M, et al. A flexible, electrochromic, rechargeable Zn//PPy battery with a short circuit chromatic warning function. J Mater Chem A 2018;6:11113-8.
56. Pandey PC, Prakash R. Electrochemical synthesis of polyindole and its evaluation for rechargeable battery applications. J Electrochem Soc 1998;145:999-1003.
57. Cai Z, Hou C. Study on the electrochemical properties of zinc/polyindole secondary battery. J Power Sources 2011;196:10731-6.
58. Cai Z, Guo J, Yang H, Xu Y. Electrochemical properties of electrospun poly(5-cyanoindole) submicron-fibrous electrode for zinc/polymer secondary battery. J Power Sources 2015;279:114-22.
59. Kye H, Kang Y, Jang D, Kwon JE, Kim B. p-type redox-active organic electrode materials for next-generation rechargeable batteries. Adv Energy Sustain Res 2022;3:2200030.
60. Qiu X, Wang N, Dong X, et al. A high-voltage Zn-organic battery using a nonflammable organic electrolyte. Angew Chem Int Ed Engl 2021;60:21025-32.
61. He W, Ren Y, Lamsal BS, et al. Decreasing water activity using the tetrahydrofuran electrolyte additive for highly reversible aqueous zinc metal batteries. ACS Appl Mater Interfaces 2023;15:6647-56.
62. Li J, Guo Z, Wu J, et al. Dextran: a multifunctional and universal electrolyte additive for aqueous Zn ion batteries. Adv Energy Mater 2023;13:2301743.
63. Dawut G, Lu Y, Miao L, Chen J. High-performance rechargeable aqueous Zn-ion batteries with a poly(benzoquinonyl sulfide) cathode. Inorg Chem Front 2018;5:1391-6.
64. Lin Z, Shi HY, Lin L, Yang X, Wu W, Sun X. A high capacity small molecule quinone cathode for rechargeable aqueous zinc-organic batteries. Nat Commun 2021;12:4424.
65. Kumankuma-Sarpong J, Tang S, Guo W, Fu Y. Naphthoquinone-based composite cathodes for aqueous rechargeable zinc-ion batteries. ACS Appl Mater Interfaces 2021;13:4084-92.
66. Kundu D, Oberholzer P, Glaros C, et al. Organic cathode for aqueous Zn-ion batteries: taming a unique phase evolution toward stable electrochemical cycling. Chem Mater 2018;30:3874-81.
67. Wang X, Chen L, Lu F, Liu J, Chen X, Shao G. Boosting aqueous Zn2+ storage in 1,4,5,8-naphthalenetetracarboxylic dianhydride through nitrogen substitution. ChemElectroChem 2019;6:3644-7.
68. Xie J, Yu F, Zhao J, et al. An irreversible electrolyte anion-doping strategy toward a superior aqueous Zn-organic battery. Energy Storage Mater 2020;33:283-9.
69. Sun T, Li Z, Zhi Y, Huang Y, Fan HJ, Zhang Q. Poly(2,5-dihydroxy-1,4-benzoquinonyl sulfide) as an efficient cathode for high-performance aqueous zinc-organic batteries. Adv Funct Mater 2021;31:2010049.
70. Mirle C, Medabalmi V, Ramanujam K. Electrode and conductive additive compatibility yielding excellent rate capability and long cycle life for sustainable organic aqueous Zn-ion batteries. ACS Appl Energy Mater 2021;4:1218-27.
71. Sun T, Zhang W, Nian Q, Tao Z. Molecular engineering design for high-performance aqueous zinc-organic battery. Nanomicro Lett 2023;15:36.
72. Buyukcakir O, Yuksel R, Begar F, et al. Ultralong-life quinone-based porous organic polymer cathode for high-performance aqueous zinc-ion batteries. ACS Appl Energy Mater 2023;6:7672-80.
73. Zhang H, Fang Y, Yang F, Liu X, Lu X. Aromatic organic molecular crystal with enhanced π-π stacking interaction for ultrafast Zn-ion storage. Energy Environ Sci 2020;13:2515-23.
74. Khayum M A, Ghosh M, Vijayakumar V, et al. Zinc ion interactions in a two-dimensional covalent organic framework based aqueous zinc ion battery. Chem Sci 2019;10:8889-94.
75. Zheng S, Shi D, Yan D, et al. Orthoquinone-based covalent organic frameworks with ordered channel structures for ultrahigh performance aqueous zinc-organic batteries. Angew Chem Int Ed Engl 2022;61:e202117511.
76. Xu D, Zhang H, Cao Z, et al. High-rate aqueous zinc-ion batteries enabled by a polymer/graphene composite cathode involving reversible electrolyte anion doping/dedoping. J Mater Chem A 2021;9:10666-71.
77. Wang Q, Liu Y, Chen P. Phenazine-based organic cathode for aqueous zinc secondary batteries. J Power Sources 2020;468:228401.
78. Liang J, Tang M, Cheng L, et al. 2,3-diaminophenazine as a high-rate rechargeable aqueous zinc-ion batteries cathode. J Colloid Interface Sci 2022;607:1262-8.
79. Li J, Huang L, Lv H, et al. Novel organic cathode with conjugated N-heteroaromatic structures for high-performance aqueous zinc-ion batteries. ACS Appl Mater Interfaces 2022;14:38844-53.
80. Li S, Shang J, Li M, et al. Design and synthesis of a π-conjugated N-heteroaromatic material for aqueous zinc-organic batteries with ultrahigh rate and extremely long life. Adv Mater 2023;35:e2207115.
81. Zhang S, Long S, Li H, Xu Q. A high-capacity organic cathode based on active N atoms for aqueous zinc-ion batteries. Chem Eng J 2020;400:125898.
82. Sun T, Zhang W, Nian Q, Tao Z. Proton-insertion dominated polymer cathode for high-performance aqueous zinc-ion battery. Chem Eng J 2023;452:139324.
83. Chen X, Su H, Yang B, Yin G, Liu Q. Realizing high-rate aqueous zinc-ion batteries using organic cathode materials containing electron-withdrawing groups. Sust Energy Fuels 2022;6:2523-31.
84. Sun G, Yang B, Chen X, et al. Aqueous zinc batteries using N-containing organic cathodes with Zn2+ and H+ Co-uptake. Chem Eng J 2022;431:134253.
85. Li J, Huang L, Lv H, et al. Investigations on the electrochemical behaviors of hexaazatriphenylene derivative as high-performance electrode for batteries. Electrochim Acta 2022;432:141206.
86. Wang W, Kale VS, Cao Z, et al. Molecular engineering of covalent organic framework cathodes for enhanced zinc-ion batteries. Adv Mater 2021;33:e2103617.
87. Wang W, Kale VS, Cao Z, et al. Phenanthroline covalent organic framework electrodes for high-performance zinc-ion supercapattery. ACS Energy Lett 2020;5:2256-64.
88. Shi Y, Wang P, Gao H, et al. π-conjugated N-heterocyclic compound with redox-active quinone and pyrazine moieties as a high-capacity organic cathode for aqueous zinc-ion batteries. Chem Eng J 2023;461:141850.
89. Sun T, Yi Z, Zhang W, Nian Q, Fan HJ, Tao Z. Dynamic balance of partial charge for small organic compound in aqueous zinc-organic battery. Adv Funct Mater 2023;33:2306675.
90. Ye F, Liu Q, Dong H, et al. Organic zinc-ion battery: planar, π-conjugated quinone-based polymer endows ultrafast ion diffusion kinetics. Angew Chem Int Ed Engl 2022;61:e202214244.
91. Huang L, Li J, Wang J, et al. Organic compound as a cathode for aqueous zinc-ion batteries with improved electrochemical performance via multiple active centers. ACS Appl Energy Mater 2022;5:15780-7.
92. Sun T, Zhang W, Zha Z, Cheng M, Li D, Tao Z. Designing a solubility-limited small organic molecule for aqueous zinc-organic batteries. Energy Storage Mater 2023;59:102778.
93. Wang Y, Wang X, Tang J, Tang W. A quinoxalinophenazinedione covalent triazine framework for boosted high-performance aqueous zinc-ion batteries. J Mater Chem A 2022;10:13868-75.
94. Huang X, Qiu X, Wang W, et al. Activating organic electrode via trace dissolved organic molecules. J Am Chem Soc 2023;145:25604-13.
95. Li W, Xu H, Zhang H, et al. Tuning electron delocalization of hydrogen-bonded organic framework cathode for high-performance zinc-organic batteries. Nat Commun 2023;14:5235.
96. Sun QQ, Sun T, Du JY, et al. In situ electrochemical activation of hydroxyl polymer cathode for high-performance aqueous zinc-organic batteries. Angew Chem Int Ed Engl 2023;62:e202307365.
97. Wan F, Zhang L, Wang X, Bi S, Niu Z, Chen J. An aqueous rechargeable zinc-organic battery with hybrid mechanism. Adv Funct Mater 2018;28:1804975.
98. Liu Z, Prowald A, Höfft O, Li G, Lahiri A, Endres F. An ionic liquid-surface functionalized polystyrene spheres hybrid electrolyte for rechargeable zinc/conductive polymer batteries. ChemElectroChem 2018;5:2321-5.
99. Tang M, Zhu Q, Hu P, et al. Ultrafast rechargeable aqueous zinc-ion batteries based on stable radical chemistry. Adv Funct Mater 2021;31:2102011.
100. Wang N, Guo Z, Ni Z, et al. Molecular tailoring of an n/p-type phenothiazine organic scaffold for zinc batteries. Angew Chem Int Ed Engl 2021;60:20826-32.
101. Zhang H, Xu D, Wang L, et al. A polymer/graphene composite cathode with active carbonyls and secondary amine moieties for high-performance aqueous Zn-organic batteries involving dual-ion mechanism. Small 2021;17:e2100902.
102. Yu P, Wang J, Gan X, Guo Z, Huang L, Song Z. Thionin as a bipolar organic cathode material for aqueous rechargeable zinc batteries. Batter Supercaps 2023;6:e202300010.
103. Yan L, Zhu Q, Qi Y, et al. Towards high-performance aqueous zinc batteries via a semi-conductive bipolar-type polymer cathode. Angew Chem Int Ed Engl 2022;61:e202211107.
104. Lu Y, Hou X, Miao L, et al. Cyclohexanehexone with ultrahigh capacity as cathode materials for lithium-ion batteries. Angew Chem Int Ed Engl 2019;58:7020-4.
105. Liang Y, Zhang P, Yang S, Tao Z, Chen J. Fused heteroaromatic organic compounds for high-power electrodes of rechargeable lithium batteries. Adv Energy Mater 2013;3:600-5.
106. Gan X, Song Z. Small-molecule organic electrode materials for rechargeable batteries. Sci China Chem 2023;66:3070-104.
107. Liao X, Pan C, Yan H, Zhu Y, Pan Y, Yin C. Polyaniline-functionalized graphene composite cathode with enhanced Zn2+ storage performance for aqueous zinc-ion battery. Chem Eng J 2022;440:135930.
108. Mandić Z, Roković MK, Pokupčić T. Polyaniline as cathodic material for electrochemical energy sources: the role of morphology. Electrochim Acta 2009;54:2941-50.
109. Zhang S, Zhao W, Li H, Xu Q. Cross-conjugated polycatechol organic cathode for aqueous zinc-ion storage. ChemSusChem 2020;13:188-95.
110. Ni Q, Kim B, Wu C, Kang K. Non-electrode components for rechargeable aqueous zinc batteries: electrolytes, solid-electrolyte-interphase, current collectors, binders, and separators. Adv Mater 2022;34:e2108206.
111. Kim J, Kim Y, Yoo J, Kwon G, Ko Y, Kang K. Organic batteries for a greener rechargeable world. Nat Rev Mater 2023;8:54-70.
112. Zhang L, Chen Y, Jiang Z, et al. Cation-anion redox active organic complex for high performance aqueous zinc ion battery. Energy Environ Mater 2024;7:e12507.
113. Cai T, Han Y, Lan Q, et al. Stable cycling of small molecular organic electrode materials enabled by high concentration electrolytes. Energy Storage Mater 2020;31:318-27.
114. Zhao Z, Lai J, Ho DT, et al. A novel “water-in-ionic liquid” electrolyte for Zn metal batteries. ACS Energy Lett 2023;8:608-18.
115. Yu L, Huang J, Wang S, Qi L, Wang S, Chen C. Ionic liquid “water pocket” for stable and environment-adaptable aqueous zinc metal batteries. Adv Mater 2023;35:e2210789.
116. Luo J, Jiang X, Huang Y, et al. Poly(ionic liquid) additive: aqueous electrolyte engineering for ion rectifying and calendar corrosion relieving. Chem Eng J 2023;470:144152.
117. Geng Y, Pan L, Peng Z, et al. Electrolyte additive engineering for aqueous Zn ion batteries. Energy Storage Mater 2022;51:733-55.
118. Li Y, Yao H, Liu X, Yang X, Yuan D. Roles of electrolyte additive in Zn chemistry. Nano Res 2023;16:9179-94.
119. Guo S, Qin L, Zhang T, et al. Fundamentals and perspectives of electrolyte additives for aqueous zinc-ion batteries. Energy Storage Mater 2021;34:545-62.
120. Naveed A, Rasheed T, Raza B, et al. Addressing thermodynamic Instability of Zn anode: classical and recent advancements. Energy Storage Mater 2022;44:206-30.
121. Ma K, Yang G, Wang C. Towards storable and durable Zn-MnO2 batteries with hydrous tetraglyme electrolyte. J Energy Chem 2023;80:432-41.
122. Ilyas F, Chen J, Zhang Y, Huang Y, Ma H, Wang J. Intrinsically safe electrolyte boosting high reversibleZn anode for rechargeable batteries. Energy Storage Mater 2023;55:566-74.
123. Li J, Ren J, Li C, et al. High-adhesion anionic copolymer as solid-state electrolyte for dendrite-free Zn-ion battery. Nano Res 2022;15:7190-8.
124. Li Y, Yuan J, Qiao Y, et al. Recent progress in structural modification of polymer gel electrolytes for use in solid-state zinc-ion batteries. Dalton Trans 2023;52:11780-96.
125. Lu K, Jiang T, Hu H, Wu M. Hydrogel electrolytes for quasi-solid zinc-based batteries. Front Chem 2020;8:546728.
126. Wu M, Su W, Wang X, et al. Long-life aqueous zinc-organic batteries with a trimethyl phosphate electrolyte and organic cathode. ACS Sustainable Chem Eng 2023;11:957-64.
127. Du W, Ang EH, Yang Y, Zhang Y, Ye M, Li CC. Challenges in the material and structural design of zinc anode towards high-performance aqueous zinc-ion batteries. Energy Environ Sci 2020;13:3330-60.
128. Hu Q, Hu J, Li Y, et al. Insights into Zn anode surface chemistry for dendrite-free Zn ion batteries. J Mater Chem A 2022;10:11288-97.
129. Bayaguud A, Fu Y, Zhu C. Interfacial parasitic reactions of zinc anodes in zinc ion batteries: Underestimated corrosion and hydrogen evolution reactions and their suppression strategies. J Energy Chem 2022;64:246-62.
130. Chen J, Zhao W, Jiang J, et al. Challenges and perspectives of hydrogen evolution-free aqueous Zn-Ion batteries. Energy Storage Mater 2023;59:102767.
131. Cao J, Zhang D, Zhang X, Zeng Z, Qin J, Huang Y. Strategies of regulating Zn2+ solvation structures for dendrite-free and side reaction-suppressed zinc-ion batteries. Energy Environ Sci 2022;15:499-528.
132. Zhang Y, Bi S, Niu Z, Zhou W, Xie S. Design of Zn anode protection materials for mild aqueous Zn-ion batteries. Energy Mater 2022;2:200012.
133. Zhu C, Li P, Xu G, Cheng H, Gao G. Recent progress and challenges of Zn anode modification materials in aqueous Zn-ion batteries. Coord Chem Rev 2023;485:215142.
134. Zheng J, Liu X, Zheng Y, et al. AgxZny protective coatings with selective Zn2+/H+ binding enable reversible Zn anodes. Nano Lett 2023;23:6156-63.
135. Zheng J, Zhu G, Liu X, et al. Simultaneous dangling bond and zincophilic site engineering of SiNx protective coatings toward stable zinc anodes. ACS Energy Lett 2022;7:4443-50.
136. Liu X, Lu Q, Yang A, Qian Y. High ionic conductive protection layer on Zn metal anode for enhanced aqueous zinc-ion batteries. Chin Chem Lett 2023;34:107703.
137. Wang C, Xu Y, Fang Y, et al. Extended π-conjugated system for fast-charge and -discharge sodium-ion batteries. J Am Chem Soc 2015;137:3124-30.
138. Luo W, Liu Y, Li F, Zhang Z, Chao Z, Fan J. Low-dimensional and high-crystallinity carbonyl cathodes prepared by physical vapor deposition for green aluminum organic batteries. ACS Appl Mater Interfaces 2023;15:37433-41.
139. Gong S, Xie Y, Zhao J, et al. An electrolyte-rich nano-organic cathode constructs an ultra-high voltage Zinc-ion battery. Chem Eng J 2023;476:146619.
Cite This Article
Export citation file: BibTeX | RIS
OAE Style
Li J, Liang H. Organic cathode materials for aqueous zinc-organic batteries. Energy Mater 2024;4:400033. http://dx.doi.org/10.20517/energymater.2023.116
AMA Style
Li J, Liang H. Organic cathode materials for aqueous zinc-organic batteries. Energy Materials. 2024; 4(3): 400033. http://dx.doi.org/10.20517/energymater.2023.116
Chicago/Turabian Style
Li, Jiahao, Hanfeng Liang. 2024. "Organic cathode materials for aqueous zinc-organic batteries" Energy Materials. 4, no.3: 400033. http://dx.doi.org/10.20517/energymater.2023.116
ACS Style
Li, J.; Liang H. Organic cathode materials for aqueous zinc-organic batteries. Energy Mater. 2024, 4, 400033. http://dx.doi.org/10.20517/energymater.2023.116
About This Article
Copyright
Data & Comments
Data
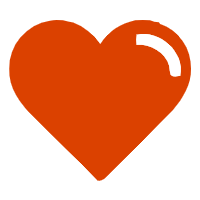

Comments
Comments must be written in English. Spam, offensive content, impersonation, and private information will not be permitted. If any comment is reported and identified as inappropriate content by OAE staff, the comment will be removed without notice. If you have any queries or need any help, please contact us at support@oaepublish.com.