Bifunctional 2D structured catalysts for air electrodes in rechargeable metal-air batteries
Abstract
The inherent technical challenges of metal-air batteries (MABs), arising from the sluggish redox electrochemical reactions on the air electrode, significantly affect their efficiency and life cycle. Two-dimensional (2D) nanomaterials with near-atomic thickness have potential as bifunctional catalysts in MABs because of their distinct structures, exceptional physical properties, and tunable surface chemistries. In this study, the chemistry of representative 2D materials was elucidated, and the comprehensive analysis of the primary modification techniques, including geometric structure manipulation, defect engineering, crystal facet selection, heteroatom doping, single-atom catalyst construction, and composite material synthesis, was conducted. The correlation between material structure and activity is illustrated by examples, with the aim of leading the development of advanced catalysts in MABs. We also focus on the future of MABs from the perspective of bifunctional catalysts, definite mechanisms, and standard measurement. We expect this work to serve as a guide for the design of air electrode materials that can be used in MABs.
Keywords
INTRODUCTION
The escalating concerns regarding energy shortages and environmental pollution arising from the overconsumption of fossil fuels are driving global technological development toward reducing fossil fuel dependence and CO2 emissions[1,2]. High-efficiency electrical energy storage is vital if we are to achieve sustainable energy, and batteries are instrumental in bridging the gap between energy collection and utilization. Although the high-energy capacity of lithium-ion batteries (LIBs) has resulted in the dominance of the consumer market, the safety issues and increasing material costs associated with these batteries remain challenging[3]. Additionally, their limited energy density hinders commercial use, especially in electric vehicles with concerns about travel range anxiety.
To circumvent these limitations, substitute energy storage and conversion devices have gained significant attention, with metal-air batteries (MABs) standing out. Of various MABs available, alkaline Zn-air batteries (ZABs) and aprotic lithium-O2 batteries (LOBs) have emerged as highly promising systems. ZABs offer a theoretical gravimetric energy density that far surpasses the current lithium-ion technology
MABs have been the subject of research since the last century; however, the development of these batteries remains nascent as compared to the well-established LIBs technologies[1]. Various challenges, including low roundtrip efficiency, poor rate performance, and limited stability, hinder the widespread adoption of MABs. Besides the impacts stemming from the electrolyte and anode, these challenges primarily arise due to the sluggish kinetics of the oxygen reduction reaction (ORR) and oxygen evolution reaction (OER) occurring at the cathode[6]. Hence, designing highly efficient bifunctional catalysts is key to the further development of MABs.
Two-dimensional (2D) nanomaterials with large specific surfaces full of exposed atoms and defects have received considerable attention as emerging catalysts[7]. Broadly speaking, 2D nanomaterials include nanomaterials with 2D morphology and 2D crystal structures. Both of them provide numerous anchor sites for atoms, maximizing the reaction efficiency and facilitating accelerated charge transport by electronic modulation. As a result, 2D materials generally exhibit superior electrocatalytic performance as compared to their three-dimensional (3D) bulk counterparts. Additionally, 2D nanomaterials serve as ideal theoretical calculation models with which to gain insights into the intricate relationship between materials structure and electrocatalytic performance, enabling exploration of the essential structure-property relationship[8,9]. The promising features of 2D nanomaterials have led to the careful design and utilization of various 2D materials as cathodes in MABs.
While several review articles have explored the possible applications of 2D nanomaterials in energy storage and conversion, a comprehensive review covering all types of 2D nanomaterials with the most commonly employed modification in both ZABs and LOBs remains unavailable. This review begins by introducing the crucial features of MABs, with a detailed explanation of the working principles behind two prominent MAB types: ZABs and LOBs. Then, we delve into the structural and property characteristics of typical 2D materials and explore various strategies for catalyst modification, including geometric structure manipulation, defect engineering, crystal facet selection, heteroatom doping, single-atom catalyst (SAC) construction, and composite material synthesis. Furthermore, relevant research on catalyst design and its influence on the overall performance of MABs are discussed, providing valuable insights into future catalyst fabrication. Finally, we emphasize the present challenges and offer perspective on the future development of efficient bifunctional catalysts for use with MABs.
OPERATION PRINCIPLES FOR AQUEOUS AND NONAQUEOUS MABs
As potential cornerstones of the future energy grid, MABs have received substantial attention over the past few decades. These batteries generally consist of four fundamental components: metal electrodes, air electrodes, electrolytes, and membrane separators. Metals that have been used as anodes in MABs include alkali metals, alkaline earth metals, and first-line transition metals (TMs) with good electrochemical equivalence. Based on the inherent reactivity of the utilized metals with water, MABs are categorized into two distinct classes: aqueous MABs (e.g., Fe, Al, Mg, and Zn) and nonaqueous MABs (e.g., Li, Na, and K)[10]. The difference in reactivity with water has led to substantial variations in their operational principles, depending on the nature of the electrolytes employed.
Mechanism of aqueous ZABs
Of the many different aqueous MABs developed so far (including Fe, Al, Mg, and Zn), ZABs have emerged as the most promising. Although Fe-air batteries possess rechargeability, their practical energy density remains significantly lower, typically falling within the range of 60-80 Wh kg-1, rendering them less competitive when compared to prevailing lithium-ion technology. These batteries find their niche in stationary energy storage applications, attributed to their extended cycle life (> 1,000 cycles), cost-effectiveness (< $100 kWh-1), and environmental compatibility[2,10]. However, their feasibility for exclusive employment in pure electric vehicle applications is limited. Al-air and Mg-air batteries exhibit considerable theoretical energy densities; however, their susceptibility to severe corrosion upon contact with aqueous electrolytes restricts their practical application. Therefore, this review focuses on ZABs as a representative case to elucidate the underlying reaction principles of aqueous MABs.
As seen in Figure 1A, the ZAB discharging process involves intricate redox reactions at both the Zn and air electrodes:
Figure 1. Schematic illustrations of device configurations and working mechanisms of aqueous ZABs and nonaqueous LOBs.
Air electrode reaction:
Zn electrode reaction:-
Overall reaction:
During discharge, atmospheric O2 molecules penetrate the gas diffusion layer and are reduced to OH- ions on the catalytic layer (equation 1). Simultaneously, Zinc ions are generated at the Zn electrode, with concomitant electron flow to the air electrode through the external circuit. The OH- ions formed at the air electrode then migrate across the electrolyte through a separator, forming into Zn(OH)42- species, which subsequently undergo dehydration to yield ZnO, as illustrated in equation 2. The overall reaction is summarized in equation 3.
It is worth noting that the ORR reaction is quite complex. Verified by many in situ characterizations, there are two possible mechanisms of ORR: a dissociation pathway and an associative pathway. In alkaline media, if the O2 molecule dissociates before the reduction, it belongs to the dissociation mechanism, going through the following steps:
where * represents the active site on the surface of catalysts.
While the O-O bond breaks after a reduction in the associative mechanism, the elementary steps proceed as follows:
And then, the reactions from O* to hydroxyl ions are the same as equations 5 and 6. The dissociation pathway is the preferred route under conditions of low oxygen surface coverage, whereas the association pathway is favored when the surface exhibits high oxygen coverage.
All of the above are four-electron transfer reactions. While a two-electron transfer ORR reaction often exists as a side reaction, which affects the efficiency of the reaction. In alkaline media, the pathway of two-electron ORR includes the following steps:
In the initial stages of the development of rechargeable ZABs, depicted reactions were confined to the forward process, characteristic of discharge. However, with the advent of bifunctional catalysts, subsequent advancements have enabled the occurrence of the reverse process, specifically, OER. The redox reactions that occur during recharge are presented as follows:
Air electrode reaction:
Zn electrode reaction:
Overall reaction:
In the charging phase, the air electrode catalyzes a four-electron transfer OER, denoted as equation 12. Concurrently, ZnO, a product from the previous discharge, undergoes reduction, reverting to its original form, Zn, as delineated in equation 13. In an ideal scenario, the charging reaction would equal the inverse of the discharge reaction, as expressed in equation 14.
Mechanism for nonaqueous LOBs
In recent decades, nonaqueous liquid alkali MABs, particularly LOBs, have emerged as prominent subjects for study due to their remarkably high-energy densities and straightforward battery architecture. The seminal work of Abraham et al. in 1996, showcasing the first rechargeable nonaqueous LOBs, marked the inception of alkali MABs[11]. LOBs, which feature Li2O2 as the discharge product, exhibit the most promising theoretical performance, boasting a high specific capacity (3,860 mA h g-1) and a cell voltage of up to
In the nonaqueous liquid system (depicted in Figure 1B), lithium metal is oxidized into a cation and subsequently shuttled through the electrolyte towards the cathode during discharge (equation 15). The desired electrochemical reaction yielding Li2O2 as the discharge product is articulated in equation 16. However, catalytic reactions typically encompass multiple steps rather than a singular equation. As demonstrated in equation 17, the intermediary LiO2 is initially formed, followed by its conversion into the final discharge product Li2O2. Furthermore, two disproportionation pathways exist for LiO2: the surface pathway (equation 18) and the solution pathway (equation 19). The solution route represents a classic electrochemical-chemical coupled reaction that was extensively explored by Savéant[12]. In instances where LiO2 disproportionates at the electrode surface, equations 18 and 19 are the same. At this stage, experimental differentiation between surface disproportionation and electro-reduction is challenging, in a manner akin to the Heyrovsky step and Tafel step competition in the hydrogen evolution reaction.
The discharging process of LOBs involves several intricate redox reactions:
Li electrode reaction:
Air electrode reaction:
The charging process, commonly recognized as the OER process, ideally involves the entirely reversible decomposition of Li2O2. However, challenges that are associated with the charging process include significant overpotential, diminished coulombic efficiency, and pronounced side reactions. These complications contribute to cathode passivation effects, voltage hysteresis, and the potential for battery deterioration over time. Experiments and theoretical calculations have yielded two primary charge reaction mechanisms for the anode surface in the nonaqueous liquid system[12]: Mechanism I, which is a two-electron process (equation 20), and Mechanism II (equations 21 and 22), which encompasses two consecutive one-electron processes that involve the formation of LiO2 from dissolved Li2O2.
When charging, air electrode reaction I:
Air electrode reaction II:
The mechanisms underlying LOBs are considerably more intricate than those associated with ZABs. Despite the preceding summary of the fundamental mechanisms of LOBs, the actual scenarios are far more complex than depicted. Consequently, a substantial array of cutting-edge in-situ research tools is required to unravel the intricate reaction mechanisms of LOBs, facilitating the design of enhanced battery systems.
STRUCTURE AND CHEMISTRY OF 2D NANOMATERIALS FOR MABs
Structure and chemistry of 2D nanomaterials
Graphene has played an instrumental role in shaping the trajectory of 2D material development. In the early years, pioneering work by Landau LD and Peierls RE indicated that strictly 2D materials exhibit thermodynamic instability at non-zero temperatures[7]. It is the discovery and characterization of 2D graphene that has led researchers to study and explore 2D materials. Presently, the integrity of the theory stands affirmed: actual 2D materials deviate from an ideal flat structure, adopting a distinctive ripple pattern. So far, dozens of 2D materials have been engineered and classified into broad categories, including elements (e.g., graphene, graphdiyne (GDY), black phosphorus (BP), and 2D metal nanosheets), nonmetallic compounds (e.g., g-C3N4, h-BN), metallic compounds [e.g., TM dichalcogenides (TMDCs), layered double hydroxide (LDH), and Mxenes], and organics [e.g., metal-organic frameworks (MOF) and covalent-organic frameworks (COF)].
Graphene, characterized by sp2 hybridized carbon atoms that are arranged in a 2D hexagonal lattice, offers advantages such as low density, large surface area, high electrical conductivity, and remarkable electrochemical stability. These attributes render graphene a cornerstone in energy storage and conversion. As exploration into graphene advances, there has been a burgeoning interest in designing carbon-based catalysts with heightened degrees of sp hybridization because it is proved that the introduction of ethynyl units may improve the electrochemical properties of carbon. The co-existence of sp-like and sp2-like carbon atoms in GDY endow them with uniformly distributed pores, high π-conjunction, and tunable electronic properties. In the past few decades, theoretical and experimental investigations unveiled the unique properties of GDY, demonstrating their potential in energy storage and conversion[13]. Different from the materials mentioned so far, the cost and air sensitivity of BP limit its viability for use in MABs. Similarly, although 2D noble metal nanosheets have demonstrated potential in laboratory settings, their low crust content hinders large-scale production, precluding their detailed discussion here.
Within the realm of nonmetallic compounds, g-C3N4 exhibits a distinctive structural composition in which the fundamental units encompass either triazine or heptazine cores that yield layered products due to their inherently planar nature. As an n-type semiconductor material, g-C3N4 has sparked considerable enthusiasm across research domains, especially in MABs, as a result of its facile synthesis, attractive electronic band structure (with conduction band and valence band energy positions at -1.1 and 1.6 eV, respectively), robust physicochemical stability, and abundance in nature[14]. Conversely, insulated h-BN, with a wide band gap, is infrequently utilized in MABs, mainly due to its lack of conductivity, which is a requirement of catalysts.
The group of 2D metallic compounds is particularly large as compared to the other three types of 2D materials. TM oxides (TMOs), hydroxides (TMOHs), sulfides (TMSs), selenides (TMSes), tellurides (TMTes), phosphides (TMPs), nitrides (TMNs), carbides (TMCs), and borides (TMBs) with nanosheet structure are all involved in this group. Many metal compounds have demonstrated prowess in catalyzing OER and ORR, with LDH, TMDC, and MXenes being the most representative. LDHs, referred to as hydrotalcite-like compounds, are a large family of 2D anionic clay materials with the formula
MOFs/COFs have emerged as the pivotal class of materials that are particularly promising for use in MABs[15]. They have garnered considerable attention due to their versatile framework structures, distinctive porosity, and expansive surface areas. MOFs comprise inorganic-organic infinite frameworks via interactions between metal nodes and organic linkers, while COFs comprise ordered honeycomb-like structures that are composed of organic linkers and linkage. While most MOFs and COFs do not inherently qualify as 2D materials, the strategic design of 2D MOFs/COFs can yield distinct advantages over their 3D counterparts. Specifically, the readily available active sites that lie on the surfaces of the exfoliated 2D MOFs/COFs layers ensure unhindered access for substrates and reagents without diffusion limitations. Another unique feature of 2D MOFs/COFs is that they have defective and exchangeable coordination positions at the metal nodes, suggesting enhanced catalytic efficiency as compared to their 3D analogs. Notably, 2D MOFs/COFs often exhibit chemical and thermal stability that is akin to or even exceeds their 3D counterparts.
Approaches to the modification of 2D catalysts
It has been widely recognized that whether it is an aqueous MAB or aprotic LOB, the performances are mainly determined by the cathode ORR and OER. Yet, both ORR and OER are hindered by substantial overpotential, sluggish kinetics, and huge energy barriers. Consequently, the development of efficient electrocatalysts assumes paramount importance. When designing and fabricating catalysts, several facets must be deliberated from structural and performance perspectives, encompassing the density of active sites, intrinsic activity of each site, electrical conductivity, robust stability, and enduring durability. Nevertheless, pristine 2D materials often lack the capacity to fulfill the multifaceted demands of a catalyst, meaning that modification and enhancement are required. In the subsequent discourse, the modification strategies for 2D materials are categorized into six principal domains: geometric structure control, defect engineering, crystal facet selection, heteroatom doping, SAC construction, and composite material synthesis [Figure 2].
Figure 2. Schematic representation of different 2D materials and approaches to mitigating the challenges associated with MABs.
The morphology control methods of 2D materials can be divided into two categories. The first pertains to the manipulation of single-layer nanosheets and includes size modulation and the introduction of holes. The second approach involves crafting hierarchical architectures that are composed of multiple nanosheets. Unlike other techniques, morphology control is aimed primarily at amplifying the number of active sites within a material to bolster its overall catalytic efficacy. Several studies have verified that cathode catalysts featuring large active surfaces are conducive to the deposition and decomposition of solid discharge products, thereby enhancing both capacity and reversibility. Furthermore, the migration of constituents such as O2, electrolyte ions, reaction intermediates, by-products, and primary discharge products within MABs directly influences the catalytic efficiency of the cathode. The constructed hierarchical structures frequently feature many voids and conduits that significantly enhance charge transfer and facilitate mass transport.
Two-dimensional materials with high surface activity provide a favorable platform for the formation and evolution of defects. Ultrathin 2D materials are believed to possess higher surface energy and abundant coordination-unsaturated sites as compared to their bulk counterparts, facilitating the development of vacancies, lattice dislocations, and distortions. Therefore, extensive investigation into defect engineering has been conducted to finely tune the physical and chemical attributes of 2D nanomaterials. Previous investigations have demonstrated that thoughtfully designed defects can proficiently modulate carrier concentration and energy band structures, thereby augmenting electrical conductivity for energy-related applications. Furthermore, a spectrum of defect engineering strategies can be harnessed to invigorate surface chemical activity and diminish reaction energy barriers within electrode materials. This approach also engenders additional active adsorption/storage sites associated with defects, thereby amplifying catalytic and electrochemical performance.
The properties of heterogeneous catalysis are intricately linked to the atomic arrangement of the exposed surfaces and geometric sites. The meticulous engineering of surface structures at the atomic level offers a means to precisely control the exposure of active sites, thereby amplifying electrocatalytic activities. Consequently, the targeted modification of material chemical and physical attributes through selective facet engineering has emerged as a pivotal research domain to optimize performances across diverse applications. Different crystal planes manifest distinct catalytic properties, including surface stability, oxygen vacancy formation energy, and interactions with surface molecules. For instance, the crystal plane effect of Co3O4 as a cathode catalyst for LOBs has garnered substantial attention in recent years. Su et al. successfully synthesized high-quality single-crystalline Co3O4 nanocrystals, including {110} facets exposed Co3O4 nanosheets, {112} facets exposed Co3O4 nanolaminars, and {111} facets exposed hexagonal Co3O4 nanoplatelets, and demonstrated that the crystal planes reduce the charge-discharge overpotential in the order: {110} < {112} < {111}, in accordance with their respective surface energy rankings[16].
Doping of 2D materials offers a means to modulate their electrical, optical, and magnetic attributes. Basically, all 2D materials are amenable to doping, and the large specific surface area with abundant exposed active sites facilitates infusion. The various techniques that can be used for doping are broadly categorized into in-situ doping during 2D material synthesis and post-doping following material preparation. Doping atoms can be both nonmetallic and metallic. Incorporating single heteroatoms, such as B, N, S, and F, can significantly reshape the electronic structure and chemical environment of active sites in 2D materials. The electron acceptance or donation between heteroatoms and the adjacent host atoms leads to altered charge distribution in the host interface, consequently influencing the adsorption energy of oxygen intermediates. In contrast to single-atom doping, multi-atom doping often engenders unexpected enhancements in catalyst performance. Dual doping within a carbon framework can yield synergistic coupling between the two heteroatoms, remarkably boosting oxygen reactivity. Metal elements can also be introduced into the structures of TM compounds as dopants to engineer tunable electrocatalytic abilities. Notably, TMs typically possess empty d orbitals that function as electrophilic sites for electrons; hence, cation doping is the suitable approach to optimizing the energy levels of catalysts for the adsorption and activation of pivotal intermediates during electrocatalysis. Moreover, the doping content significantly influences the catalytic performance of 2D nanomaterials, mirroring the alteration extent in the intrinsic host material structure. To a certain degree, increasing the doping content leads to a rise in the number of unsaturated sites in TM compounds, augmenting electrocatalysis. However, excessively high dopant content can also trigger undesirable effects in both structural and physical properties. The reduced distance between dopants associated with excessive doping can potentially lead to aggregation and even the formation of secondary phases. Therefore, when designing metal-doped catalysts, special attention should be paid to the doping content.
The concept of single-site heterogeneous catalysts was first proposed by John in 2005 when development was initiated with the first preparation of a single-atom Pt catalyst on FeOx[17,18]. Researchers have discovered that reducing the size of bulk catalysts to a single atom (SA) on a substrate conserves the usage of metal sources and leads to an alteration of their electronic state from a continuous one to discrete features, potentially yielding unique properties. Recent investigations have validated unexpectedly excellent characteristics for SAs loaded onto ultrathin nanosheets as compared to larger-size nanoparticles loaded onto 2D nanosheets or SAs loaded onto 3D bulk carriers. Characteristic SACs that are loaded onto 2D materials exhibit the following traits. First, when compared to 3D materials that support SACs, the SAs confined within 2D structures tend to possess more coordinatively unsaturated states due to vacancies on both sides of the basal plane. This unsaturation renders them more likely to achieve enhanced catalytic performance. Second, the open structure on both sides of the 2D plane facilitates a rapid mass transfer process as compared to SACs on 3D supports, theoretically ensuring the complete exposure of SAs to reactants and maximizing catalytic reaction rates. Third, advanced characterization techniques can accurately probe the local atomic structure and electronic states of the SAs that are confined within 2D materials, not only enabling prediction of the molecular reaction dynamics during catalysis but also facilitating model studies that can investigate the intricate interplay between geometric effects, electronic effects, and catalytic performance. It is worth noting that confined SAs can be either exposed directly for use as active sites on 2D supports or embedded within 2D structures, where they modify the electronic properties of inherently inactive 2D materials to initiate catalytic activity. The strong electronic interaction between confined SAs and the 2D structure fundamentally impacts the catalytic properties of an active site. In essence, precise catalyst control can be attained by manipulating the electronic structure of a SA or the surface chemical environment of a 2D carrier. Taking the example of a SA on graphene, it features atomically dispersed TM centers (e.g., Fe, Co, Ni, Cu, Mn) and their adjacent coordinated atoms confined within carbon frameworks. Additionally, heteroatoms such as N, S, O, and P can be doped into the carbon matrix, referred to as environmental atoms. Moreover, the central metal atoms have unsaturated coordination and can interact further with potential guest groups, including small molecules and inorganic particles. Recent research has also explored the introduction of additional central metal atoms into the system, with the newly developed multi-atom catalysts exhibiting higher intrinsic activity due to the synergistic effects between the adjacent central metal atoms. It should be noted that the construction of SACs does have similarities with metal doping, a fact that can be confusing. When it comes to the definition, doping is the introduction of foreign atoms into the lattice of catalysts, in which the main point is the location of heteroatomic doping. However, the focus of SACs is whether the introduced heteroatom is surrounded by the same metal atom. When the amount of doping is small, the introduced metal atoms can be dispersed separately in the catalyst, and the resulting material can be considered as the SAC. As the content of metal dopants increases, the metal atoms are difficult to isolate, so the catalyst is not the SAC anymore.
Constructing complexes is also a common strategy for enhancing catalyst performance. Since a single material generally cannot simultaneously fulfill the activity, conductivity, and stability requirements, the introduction of another material becomes essential. This is particularly significant in MABs where catalysts need to efficiently facilitate both OER and ORR. Finding an electrocatalyst with high activity for either ORR or OER is usually achievable; however, discovering one that has high activity for both reactions is challenging due to the distinct demands for different active sites or metal-ion valence states. Numerous heterostructures of varying dimensionalities have been synthesized for MABs, including zero-dimensional (0D) nanoparticles loaded onto 2D nanosheets, one-dimensional (1D) nanotubes or nanofibers incorporated into 2D nanosheets, and 2D nanosheets combined with other 2D nanosheets. Developing heterostructures based on 2D materials not only retains the inherent advantages of pristine 2D materials but also can introduce new possibilities to properties of materials such as exceptional mechanical strength, rapid electronic and ionic conductivities, enhanced electrochemical activity, and structural stability.
2D MATERIALS FOR AQUEOUS ZABs
ZABs have gained attention as potential sustainable energy solutions thanks to their notable energy density, cost-efficiency, and safety attributes. However, the dearth of suitable bifunctional catalysts poses a notable hindrance to the progress of ZABs. Among the many alternative materials, 2D materials with large surface area, controllable structure, and unique physicochemical promise to act as high-efficiency bifunctional catalysts in ZABs[19]. 2D materials, such as graphene, GDY, TMOs/TMOHs, TMDCs, TMPs, MXenes, BP, COFs, and MOFs, have been increasingly utilized in OER and ORR, especially in the last five years[20]. Despite the fact that the substantial effects of advanced 2D materials have contributed to the development of ZABs, this field of research is still in its infancy. Therefore, it is necessary to summarize the recent advances of various 2D materials in ZABs. Here, we divide 2D nanomaterials into five broad categories. The detailed electrochemical performance, including the overpotential of OER (Ej = 10), the half-wave potential of ORR (E1/2), power density, and specific capacity in ZABs, were briefly compared in Table 1. Also, for easier comparison of various catalysts, OER and ORR performance are listed in Figure 3. Then, detailed descriptions of each of these materials applied in ZABs were given below.
Comparison of different types of 2D electrocatalyst performance for ZABs
Electrocatalysts | OER | ORR | ZABs | Refs. | ||||||
Ej = 10 (V vs. RHE) | E1/2 (V vs. RHE) | Electrolyte | Peak power density (mW cm-2) | Specific capacity (mAh gZn-1) | Cycling stability | |||||
2D Carbon-based materials | NGM | 1.67 | 0.77 | 6 M KOH | 3 | - | - | [21] | ||
d-pGCS-1000 | - | 0.82 | 6 M KOH + 0.2 M Zn(Ac)2 | 182.8 | 773@5 | - | [22] | |||
NCF | - | 0.85 | 6 M KOH + 0.2 M Zn(Ac)2 | 173 | - | - | [23] | |||
N/biochar-800-7 | - | 0.9 | 6 M KOH | 125 | 703@5 | 60 h at 5 mA cm-2 | [24] | |||
N-GDY-900 | 1.796 | 0.83 | 6 M KOH | 84 | 693@10 | Over 300 h (450 cycles) | [25] | |||
2D-PPCN-2/6 | - | 0.85 | 6 M KOH + 0.2 M Zn(Ac)2 | - | - | 1,000 cycles | [26] | |||
NPC-“Li” | 1.559 | 0.83 | 6 M KOH + 0.2 M Zn(Ac)2 | - | 733@20 | 10 min/cycle for 150 cycles | [27] | |||
NSP-Gra | 1.76 | 0.82 | 6 M KOH | 225 | - | 40 h | [28] | |||
NPS-G | - | 0.857 | 6 M KOH + 0.2 M Zn(Ac)2 | 151 | 686@10 | 20 h at 10 mA cm-2 | [29] | |||
Metal-decorated 2D carbon materials | Co@NCNSs-900 | 1.59 | 0.85 | 6 M KOH + 0.2 M Zn(Ac)2 | 271 | - | 400 cycles (133 h) | [30] | ||
CuCo@N-C | 1.46 | 0.81 | 6 M KOH + 0.2 M Zn(Ac)2 | 170 | 822@5 | 300 cycles (about 50 h) | [31] | |||
Fe-N/C-700 | - | 0.84 | 6 M KOH | - | 727@5 | 100 h at 5 mA cm-2 | [32] | |||
FeNC-D0.5 | - | 0.866 | 6 M KOH | 356 | - | 25 h at 10 mA cm-2 | [33] | |||
FeMn-DSAC | - | 0.922 | 6 M KOH + 0.2 M Zn(Ac)2 | 184 | - | 200 cycles (80 h) | [34] | |||
Zn-SAs/UNCNS | - | 0.91 | 6 M KOH | 282 | 798.6@100 | Over 20 h at 10 mA cm-2 | [35] | |||
SA-Fe-SNC@900 | 1.632 | 0.876 | 6 M KOH + 0.2 M Zn(Ac)2 | 218.6 | 798.7@5 | 200 h at 10 mA cm-2 | [36] | |||
Fe-N, O/G | - | 0.86 | 6 M KOH | 164.7 | - | >150 h at 20 mA cm-2 | [37] | |||
A-Fe-NC | - | 0.865 | 6 M KOH + 0.2 M Zn(Ac)2 | 132.2 | - | 240 h at 10 mA cm-2 | [38] | |||
CoSA/NCs | 1.533 | 0.87 | 6 M KOH + 0.2 M Zn(Ac)2 | 255 | 908.7@20 | 6,000 cycles (~ 2,000 h) | [39] | |||
Fe-SA-NSFC | - | 0.91 | 6 M KOH + 0.2 M Zn(Ac)2 | 247.7 | 792.1@10 | 240 h at 10 mA cm-2 | [40] | |||
YN4-Cl | - | 0.85 | 6 M KOH + 0.2 M Zn(Ac)2 | 162 | 770@200 | 20 h at 20 mA cm-2 | [9] | |||
Zn-N4-O | - | 0.884 | 6 M KOH + 0.2 M Zn(Ac)2 | 182 | 796.6@100 | 160 h at 10 mA cm-2 | [41] | |||
O-PdN4-NGS | - | 0.90 | 6 M KOH + 0.2 M Zn(Ac)2 | 178 | 793@10 | 240 h at 10 mA cm-2 | [42] | |||
2D transition metal oxide/hydroxide | Ni-doped CoO NSs | - | - | 6 M KOH | 377 | 655@30 | > 400 h at 5 mA cm-2 | [43] | ||
N/P-Cu0.1Co0.3Mn0.6O2/CNTs | 1.52 | 0.82 | 6 M KOH + 0.2 M Zn(Ac)2 | 108.1 | - | > 200 h at 10 mA cm-2 | [44] | |||
ODAC-CoO-30 | 1.549 | 0.849 | 6 M KOH + 0.2 M Zn(Ac)2 | 128.5 | 705.6@20 | 150 h at 5 mA cm-2 | [45] | |||
porous Ni/NiO | 1.49 | 0.76 | 6 M KOH + 0.2 M Zn(Ac)2 | 225 | 853@20 | 120 h at 2 mA cm-2 | [46] | |||
NiCo2O4-rGO | 1.61 | 0.78 | 6 M KOH + 0.2 M Zn(Ac)2 | 125.3 | 719.8@5 | 200 cycles at 5 mA cm-2 | [47] | |||
NiCo2O4 nanosheet | - | 0.85 | 6 M KOH + 0.2 M Zn(Ac)2 | 102.08 | - | 29 h at 10 mA cm-2 | [48] | |||
NiCo2.148O4 PNSs | 1.42 | - | 6 M KOH | 83 | - | 120 h at 2 mA cm-2 | [49] | |||
PBMNC/LDH-20 | 1.66 | - | 6 M KOH + 0.2 M Zn(Ac)2 | 65.5 | 695.6@10 | 100 h at 5 mA cm-2 | [50] | |||
S-LDH/NG | 1.474 | 0.692 | 6 M KOH + 0.2 M Zn(Ac)2 | 165 | 772@5 | 120 h at 5 mA cm-2 | [51] | |||
Other 2D transition metal compounds | CoP@CC | 1.53 | 0.67 | 6 M KOH + 0.2 M Zn(Ac)2 | 30 | - | 10 h at 10 mA cm-2 | [52] | ||
Co9S8/FeNC | 1.58 | 0.865 | 6 M KOH + 0.2 M Zn(Ac)2 | 214.7 | 780.7@5 | 300 h at 5 mA cm-2 | [53] | |||
Co3FeN | 1.65 | 0.86 | 6 M KOH | 108 | 798@5 | 150 h at 5 mA cm-2 | [54] | |||
Re-Ni3S2/NG/NF | 1.477 | 0.66 | 6 M KOH + 0.2 M Zn(Ac)2 | 99 | 731.1@5 | 833 h at 5 mA cm-2 | [55] | |||
FePSe3-O Ns | 1.491 | 0.797 | 6 M KOH + 0.2 M Zn(Ac)2 | 38 | - | 280 h at 10 mA cm-2 | [56] | |||
Pt/NBF-ReS2/Mo2CTx | 1.564 | 0.911 | 6 M KOH + 0.2 M Zn(Ac)2 | 117.3 | 786@50 | 100 h at 5 mA cm-2 | [57] | |||
Other 2D catalysts | BHZ-48 | 1.40Ej= 50 | 0.84 | 6 M KOH + 0.2 M Zn(Ac)2 | 148 | - | 1,250 h at 5 mA cm-2 | [58] | ||
CFZ | 1.50 | 0.82 | 6 M KOH + 0.2 M Zn(Ac)2 | 178 | - | 500 h at 25 mA cm-2 | [8] | |||
FeNi LDH-TpFx | 1.84 Ej= 100 | - | 6 M KOH + 0.2 M Zn(Ac)2 | 118 | 798@139 | 800 cycles at 5 mA cm-2 | [59] | |||
Co-CNNs-0.7 | 1.67 | 0.803 | 6 M KOH + 0.2 M Zn(Ac)2 | 85.3 | 675.7@10 | 183 h at 10 mA cm-2 | [60] | |||
Co-NC-900 | - | 0.838 | 6 M KOH + 0.2 M Zn(Ac)2 | 160 | - | 45 h at 10 mA cm-2 | [61] | |||
BP-CN-c | 1.66 | 0.84 | 6 M KOH + 0.2 M Zn(Ac)2 | 168.3 | 793.9@5 | 300 h at 10 mA cm-2 | [62] |
2D carbon-based materials
Carbon materials are employed as conductive additives and electrocatalysts for ZABs owing to their excellent conductivity, cost-effectiveness, and customizable structure[63]. However, the thermodynamically low corrosion potential of pure carbon (+0.207 V vs. NHE) can induce electrochemical corrosion during the cell operation. It will further accelerate the deactivation of catalytic active sites due to the production of some stable oxidative intermediates, such as phenols, lactones, and carboxylic acids, and finally lead to a decline in both the activity and durability of carbon-based materials. To solve the carbon corrosion issue at high anodic potentials, some strategies can be employed, including the modification of its composition utilizing heteroatom-doped carbon, the synergistic cooperation incorporating carbon with other materials via a strong coupling interaction, and the manipulation of the crystal structure enhancing graphitization and reducing defect[64,65]. In recent years, the 2D graphitic structures, such as graphene and GDY, have been extensively utilized for enhancing the electrocatalytic stability because their tightly packed sp2 hybridized carbon bonds possess the robustness against the corrosion during the electrochemical cell operation[66,67]. While 2D carbon-based materials play a vital role as cathode materials in ZABs, there remains a substantial distance in catalytic activity when compared to metal-based materials. So, it is necessary to enhance the catalytic activity, mainly strategies including defect engineering (e.g., topological defects and edges) and heteroatom doping (N, P alternating electron and active sites)[68].
Early research on modifying carbon materials primarily emphasized surface functionalization or geometric structuring. As a result, the significance of carbon defects is frequently overlooked. Topological defects in carbon-based materials are inevitable, arising from fabrication or occurring naturally due to crystal disorder[69-71]. Several researchers have verified that introducing topological defects into the carbon framework disrupts the distribution of delocalized orbitals within the initial hexagonal lattice. Theoretical computation and experimental investigations have elucidated that manipulating the electronic structure of the carbon matrix influences its binding affinity and electrochemical performance[72,73]. Tang et al. conducted comprehensive research into the theoretical contribution of topological defects to ORR and OER performances, using free energy diagrams and pertinent overpotentials as the foundation of their study[21]. Specifically, using natural gelatinized sticky rice as raw material, a graphene catalyst with rich topological defects was designed and synthesized, as seen in Figure 4A. The results revealed that for simplex defect sites, the pentagon-heptagon pair exhibited the lowest overpotential, with the pentagon following closely, surpassing all nitrogen species in terms of performance. Furthermore, the researchers constructed primary ZABs to explore the practical viability of the synthesized nitrogen-doped graphene mesh (NGM). The ZAB with a prepared air electrode exhibited a high open circuit voltage (OCV) of
Figure 4. (A) (a) TEM image of graphene layer; (b) LSV curves of ORR for the catalyst at different electrolytes; (c) Polarization and power density curves of ZABs[21]. Copyright 2016, Wiley-VCH. (B) (a) SEM images of N-GDY-900; (b) ORR and OER performance of four catalysts compared with commercial Pt/C and IrO2; (c) Charge and discharge polarization curves of N-doped GDY[25]. Copyright 2021, Elsevier. (C) (a) SEM image of 2D-PPCN; (b) ORR activity of the prepared samples; (c) GCD polarization curves of 2D-PPCN and Pt/C+Ir/C based air electrode[26]. Copyright 2018, American Chemical Society. (D) (a) TEM image of NSP-Gra; (b) ORR and OER performance of the catalysts; (c) Polarization and power density curves of the primary ZABs[28]. Copyright 2021, Elsevier.
Edge and topological defects dominate the inherent imperfections of carbon materials and are widely accepted as pivotal active sites for ZABs[72,74]. Owing to the distinct bonding configurations that distinguish edge sites from basal planes, edges typically show diverse electrochemical and thermodynamic characteristics. Tao et al. reported edge-rich and dopant-free graphene[75], demonstrating the crucial role of edge carbon in enabling efficient ZABs without the requirement for external dopants. Additionally, it is noteworthy that of the various types of nanocarbon, 2D carbon nanostructures indisputably offer the most abundant number of edge sites for nitrogen doping. Nevertheless, the edge area of 2D nanocarbons requires further enhancement compared to the basal planes. Consequently, the ORR contribution, which originates in nitrogen in the basal configuration, cannot be extracted, leading to difficulties in assessing the genuine nitrogen-based ORR activity at the edges. In response to these challenges, Zhang et al. introduced a creative approach combining graphitization with nitrogen modification[23]. This strategy effectively yielded novel metal-free carbon nanoflakes (NCF) with enriched edges and a similar structure to graphite[23]. Benefiting from the synergistic effect of edge-type pyridinic-N/graphitic-N dipole, NCF exhibited high ORR performance with a half-wave potential of 0.85 V. The assembled ZAB delivered a stable OCV of 1.41 V and a high peak power density of 173 mW cm-2.
Doping is widely acknowledged as an effective strategy for enhancing the intrinsic site activity of carbon-based oxygen electrocatalysts. The method can increase the number and improve the activity of active sites via geometric, electronic, and synergistic influences. Compared to carbon atoms, heteroatoms' distinct radii and electronegativity mean that their incorporation within the carbon framework can activate charge distribution and spin density in the surrounding carbon matrix[76]. Due to their manifold benefits, nitrogen atoms have gained significant attention from diverse heteroatoms. N-doped carbon nanotubes (CNTs) as catalysts for the ORR were reported in 2009, with notably superior activity compared to pristine CNTs[77]. Since then, numerous studies have been carried out in this direction. For example, Ma et al. obtained N-doped biochar material (N/biochar) via liquid-assisted carbonization with microalgae biomass as the precursor[24]. The optical N/biochar exhibited remarkable ORR activity with E1/2 of 0.90 V due to the active pyridinic N species. Furthermore, the prepared ZAB with N/biochar demonstrated impressive specific capacity (703 mAh gZn-1) and high stability (60 h). To elucidate the source of this enhanced performance, density functional theory (DFT) calculations were performed. The results suggest that pyridinium-N atoms can modify the band structure of neighboring carbon atoms, thereby facilitating the dissociation of O2 molecules onto neighboring carbon atoms. After that, Lu et al. successfully synthesized nitrogen-doped GDY (N-GDY-900) with pyridinic N as its main nitrogen doping form via a facile pyrolysis route at 900 °C [Figure 4B][25]. As a result, the ZAB assembled by N-GDY-900 delivered a high OCV of 1.54V and improved stability for 300 h (450 cycles). DFT calculations have further shown that the N-atoms forming the pyridine configuration in GDY can induce a higher positive charge density on the ortho-carbon atoms, resulting in a lower energy barrier. In addition to nitrogen atoms, carbon materials doped with atoms such as B, S, and P also exhibit favorable electrocatalytic activity in oxygen reactions[78-80]. Lei et al. synthesized 2D P-doped porous carbon nanosheets (2D-PPCN) through the multifunctional templating approach [Figure 4C][26]. Benefiting from the P doping and porous structure, the ZAB of 2D-PPCN exhibited remarkable performances, including a high OCV (1.40 V) and excellent cycling stability for more than 1,000 charge-discharge cycles. The multiple doping strategy imparts a synergistic influence that further augments the electrocatalytic prowess of metal-free heteroatom-doped carbon catalysts. Both theoretical calculations and experimental studies have verified that incorporating nitrogen atoms into carbonaceous materials can enhance the conductivity of carbon via electron donation by shifting the Fermi level toward the conduction band[81]. A second nonmetallic heteroatom, such as P, S, or F, associated with N-doped carbons (NCs), can further modulate the electronegativity and surface polarities, significantly elevating ORR performance. Recently, doping with heteroatoms modulates the electronic surface of carbon and fine-tunes the catalytic activity (such as S and N[82], P and N[83-85]) have been broad investigations. For instance, Li et al. synthesized N, P co-doped hydrophilic carbon nanosheets (NPC-“Li”)[27]. The obtained NPC-“Li” exhibited exceptional catalytic performance for both ORR and OER, attributed to the synergistic effect of N and P co-doping with the resulting electronic interactions conducive to the catalytic process. More importantly, ZABs with NPC-“Li” as the cathode demonstrated a high specific capacity (908 Wh kgZn-1) and long-time cycle stability
Metal-decorated 2D carbon materials
At present, the development of low-cost metal catalysts to replace traditional commercial Pt/C applications in aqueous ZABs is an inevitable way to achieve the widespread popularity of ZABs. Recently, it has been reported that abundant and cost-effective TMs, such as Ni, Mn, Co, Fe, and Mo, exhibit promising catalytic activities for both the OER and ORR. Furthermore, TM-based catalysts exhibit remarkably similar catalytic activity to noble metal-based catalysts and demonstrate significantly enhanced corrosion resistance compared to metal-free catalysts. While challenges such as low intrinsic activity, limited conductivity, and inadequate stability persist, their relatively strong catalytic performance and exceptional selectivity render them promising candidates for bifunctional electrocatalysts and even potential applications in ZABs[88-90]. Thus, considerable efforts have been directed towards TM-based catalysts in the past decade. According to the geometric size reduction principle to increase the number of active sites, the research of metal nanoparticles, clusters, and SA catalysts has been greatly developed.
Metal nanoparticles or alloy-modified carbon nanomaterials also demonstrate exceptional electrocatalytic activity, promoting the development of ZABs[91-93]. Zheng et al. synthesized a hybrid of NC nanosheets encapsulating Co nanoparticles (Co@NCNSs)[30]. The optimized hybrids Co@NCNSs-900 demonstrate excellent catalytic performances in OER and ORR due to the high surface area and the synergistic effect between Co nanoparticles and NC nanosheets. As a result, the assembled ZAB of Co@NCNSs-900 showed a higher power density (271 mW cm-2), and no degradation was found after 400 cycles. The synergistic effect between metal nanoparticles can regulate the local electron density of the catalyst and produce abundant active sites. As shown in Figure 5A, a bifunctional electrocatalyst with ultrahigh activity, composed of Cu and Co nanoparticle-co-decorated N-doped graphene nanosheets (CuCo@N-C), were synthesized through a template-free strategy by Liu et al.[31]. The CuCo@N-C possesses good ORR activity (E1/2 = 0.81 V) and an outstanding overpotential of 1.46 V at the current density of 10 mA cm-2, indicating the strong electron transfer between Cu and Co enhanced reaction kinetics, promoting catalytic activity. Meanwhile, when CuCo@N-C was employed as the cathode, the prepared ZAB presented a high open circuit potential
Figure 5. (A) (a) TEM image of CuCo@N-C; (b) ORR and OER curves of CuCo@N-C, Co@N-C, Cu@N-C and N-C; (c) Charge-discharge curves of Pt/C and CuCo@N-C[31]. Copyright 2019, the Royal Society of Chemistry. (B) (a) SEM image of Fe-N, O/G; (b) LSV tests curves of the ORR for the Fe-N,O/G and other electrocatalysts; (c) Discharge polarization curves and power densities[37]. Copyright 2023, the Royal Society of Chemistry. (C) (a) HAADF-STEM image the Fe-SA-NSFC; (b) ORR polarization curves of the Fe-SA-NC, Fe-SA-NSC, Fe-SA-NSFC, and Pt/C; (c) Discharge polarization curves and power density curves[40]. Copyright 2020, Springer Nature.
Following the principle of enhancing active sites through a geometric size reduction, SACs offer the potential to expose a wealth of active sites, maximizing atomic utilization and displaying distinctive physicochemical properties[97,98]. A representative study by Qiao et al. in 2011 involved the atomic dispersal of Pt atoms onto iron oxide, where they catalyzed the CO oxidation reaction[18]. The proposed SACs exhibit unique merits, such as maximum atomic efficiency across the entire catalytic process. Since then, substantial efforts have been dedicated to synthesizing and utilizing the family of SACs.
In developing SACs, significant effort has been devoted to enlarging noble metals' atomic utilization efficiency and achieving efficient catalytic activity in high-performance ZABs. Most SACs designed as oxygen electrocatalysis are anchored onto nitrogen-doped carbon-based materials with a typical M-N-C structure[99]. Several studies have demonstrated that regulating the guest groups and the central metal, coordination, and environmental atoms can significantly enhance the intrinsic electrocatalytic activity of
Usually, when tuning the intrinsic catalytic activity of SACs, the most direct and effective strategy is to select a suitable central metal atom. For example, during the ORR process, the d orbitals of the central metal atom can interact with the p-orbital electrons of the oxygen intermediate, facilitating the adsorption and decomposition of O2 molecules. However, achieving precise control over the structure of SACs has proved difficult, and the direct derivation of SACs with excellent ORR electrocatalytic activity is challenging. To address this problem, a novel theoretical simulation approach to the ORR, pioneered by Nørskov et al., provided a convenient way to compare the intrinsic ORR activities of SACs under different center metal atoms[100]. The intrinsic ORR activity was proved by Peng and Zheng et al. The experiment with theoretical calculation indicated that follows the sequence Fe > Co > Cu > Mn > Ni[101,102]. For example, Yang et al. obtained highly active self-supporting iron-coordinating nitrogen-doped carbon catalysts (Fe-N/C) through pyrolysis of the iron coordination complex precursor[32]. The optimized catalyst Fe-N/C-700 exhibits a half-wave potential (E1/2 = 0.84 V vs. RHE) for the ORR. Besides, the ZAB with the Fe-N/C-700 as the cathode catalyst shows a high specific capacity of 727 mAh g-1 and an energy density of 965 Wh kg-1 at 5 mA cm-2. To obtain more active Fe-N4 sites, Shao et al. achieved petal-like porous carbon nanosheets with densely accessible Fe-N4 sites through the pyrolysis of a novel iron complex derived from diethylenetriaminepentaacetic acid (DTPA) and melamine (FeNC-D)[33]. The optimal catalyst FeNC-D0.5 has numerous active sites and can enable exposure to more originally inaccessible sites due to the special fluffy petaloid nanosheet structure. Furthermore, the FeNC-D0.5-based ZAB can achieve a remarkable power density of 356 mW cm-2. Cui et al. successfully obtained a catalyst with Fe-Mn dual-metal sites anchored on ultrathin 2D NC nanosheets with a porous structure (FeMn-DSAC)[34]. The synergistic effect of the Fe-Mn dual-sites can enhance active site exposure and thus improve mass transfer over the porous 2D nanosheet structure. FeMn-DSAC was found to exhibit exceptional bifunctional activity, enabling ZABs to function efficiently even at ultra-low temperatures of -40 °C, with an ultrahigh peak power density of
The coordination atoms (such as N, C, and O) can directly bond with the central metal atoms during synthesis. Therefore, regulating the ligand structure can directly affect the central metal atom and thus can promote/inhibit the performance of OER and ORR. Specifically, modifying the coordination structure by altering the coordination number and atoms present can lead to substantial changes in the electronic configuration of the central atom. The efficacy and simplicity of this approach have been progressively demonstrated in the enhanced ORR/OER activity of SACs[103-105]. For example, Zhang et al. studied the effect of the coordination environment of single Zn atoms on the ORR activity by constructing single Zn atoms on ultrathin 2D NC nanosheets (Zn-SAs/UNCNS) via pyrolysis of Zn-tetrakis(4-carboxyphenyl)porphyrin nanosheets at 900 °C[35]. DFT calculations demonstrated that the electronic states of the divacancy
In addition to replacing N atoms, changing the coordination number of the central metal atoms has been identified as a viable approach to directly regulating the electronic structure of a metal atom toward efficient catalytic activity[106-108]. Shang et al. adopted a "coordination design strategy" to prepare a catalyst (A-Fe-NC) by anchoring single-atom Fe on nitrogen-doped carbon nanosheets[38]. X-ray absorption spectroscopy revealed a coordination number of 5 for the Fe atom, suggesting that five N coordination atoms are coordinated with the central Fe atom. Benefiting from the Fe-N5 and the unique 2D sheet structure, the flexible ZABs based on the well-designed catalyst exhibit a peak power density of 132.2 mW cm-2 and significant charge-discharge cycling stability (240 h). Li et al. constructed bifunctional CoN3 catalytic sites, which present a template method that was dispersed onto N-doped graphitic carbon nanosheets (CoSA/NCs)[39], with the experimental and theoretical modeling results confirming that the single-atomic dispersed CoN3 has better catalytic activity than CoN4. The CoSA/NCs-based ZABs show a significant peak power density (255 mW cm-2) and excellent long-term stability. Modifying the coordination structure of SACs thus plays a crucial role in effectively manipulating the electronic structure of the central metal atoms.
Environmental atoms are substrate atoms that do not establish direct bonds with central metal or ligand atoms. The planar arrangement of active sites and carbon substrates induces significant long-range interactions among the d/p electrons of various atoms within distinct domains. Consequently, incorporating heteroatoms such as S, B, or P into the carbon-based substrate can indirectly impact catalytic activity. Zhou et al. presented a method to anchor a SA in nitrogen-, sulfur- and fluorine-co-doped graphitized carbons (M = Fe, Co, Ru, Ir, and Pt) via an effective ligand-stabilized pyrolysis strategy. Among them, Fe-N-Cs with S and F-co-doping (Fe-SA-NSFC) electrocatalysts demonstrated the highest ORR activity with a positive half-wave potential of 0.91 V, as shown in Figure 5C[40]. S, F co-doping significantly decreases the free energy barrier on the FeN4 active sites. Therefore, Fe-SA-NSFC shows a good performance in ZABs, with a large peak power density of 247.7 mW cm-2 and long-term stability over 240 h. In others, Li et al. used high-temperature pyrolysis to synthesize single-atom iron-loaded boron-nitrogen-doped carbon catalysts (Fe-N4-B)[109]. The as-designed Fe-N4-B SAC exhibited high ORR activity with a half-wave potential of 0.859 V. Additionally, the notable bifunctional activity achieved by Fe-N4-B can be attributed to the effective modulation of the d-band Fe center through B atoms, which optimizes the adsorption behavior of oxygenated species.
Guest groups can be introduced to interact with the central metal atoms in the M-N-C SAC host, effectively acting as ligands that coordinate with the active sites of the SAC. Generally, the d orbitals of the central metal atoms oriented perpendicular to the M-N-C plane are often incompletely occupied, allowing for potential interactions with small-molecule ligands. Consequently, external ligands can coordinate with central metal atoms, thereby regulating the electronic structures of M-N-C sites and modulating intrinsic ORR and OER activity. For instance, chloride ions possess strong coordination capabilities with TM atoms. Ji et al. introduced Cl ions to modify the original yttrium single-atom (YN4) active sites[9], and the resulting YN4-Cl moieties embedded within the carbon framework displayed a notable half-wave potential of 0.85 V in ORR, delivering a peak power density of 162 mW cm-2 and long-term stability in ZABs. The chlorine atom serves as a suitable axial ligand, forming a YN4-Cl covalent bond with a moderate coupling between its 3p orbital and the 4d orbital of Y. This bond adapts to elongate self-adaptively, aiding in facilitating intermediate binding during the ORR process. Similarly, M-N-C, with M-N4 sites modified by
2D transition metal oxide/hydroxide
The abundant availability of TMOs and TMOHs holds substantial promise for enhancing the performance of rechargeable ZABs. Currently, catalysts based on precious metals or metal oxides, such as Pt, IrO2, or RuO2, have been extensively explored in ZABs. However, the scarcity and high costs of these materials restrict the broad utilization of these catalysts. Numerous cost-effective alternatives using earth-abundant materials have been devised to address these challenges and enable the widespread implementation of ZABs. In the ZABs, both OER and ORR activity should be considered in the selection of matrix metal oxides. In catalyzing oxygen evolution, ΔGO-ΔGOH was widely employed as the descriptor of metal oxide activity[111]. Out of the precious metal-based oxides, FeNi or FeCo bimetallic oxides show the best values and are listed at the top of the volcanic plot. Experiments also bore out this view according to Table 1. However, in the ORR, there are few such widely accepted theoretical studies for metal oxides. According to the recent reports, the commonly used oxide-based ORR catalysts mainly include Co- and Mn-based oxides. In the practical approach, not only the choice of metal oxide for the catalysts but also the modification of their functional activities should be carried out to achieve high-performance bifunctional catalysts with excellent performance and stability.
Cobalt oxides (CoOx) are promising for advancing ZABs due to their remarkable bifunctional catalytic capabilities, particularly the spinel-type oxide Co3O4[112,113]. Typically, the tetrahedral sites in Co3O4 are occupied by Co2+ ions, while the octahedral sites host Co3+ ions[114]. Catalysts that feature a surface enriched with Co2+ ions have been found to display improved ORR performance, while OER activity benefits from the presence of more Co3+ sites on the surface. This heightened catalytic activity can be attributed to the enhanced adsorption of O2 and OH- at the Co2+ and Co3+ sites, respectively[115]. For instance, He et al. synthesized an ultrathin Co3O4 nanofilm that was only approximately 1.8 nm thick[116]. The ultrathin nanosheets or nanofilms altered the adsorption/desorption behaviors of the oxygen-containing species, enhanced the intrinsic conductivity, and dramatically promoted the reaction kinetics. Enriching the 2D nanostructure surface with Co3+ ions promoted the kinetics of the catalytic reaction. Moreover, the Co3O4 nanofilm enabled the production of rechargeable ZABs with a low overpotential of 0.72 V, higher round-trip efficiency (62.7%), and excellent cycling stability (175 cycles).
Despite the various advantages of Co3O4 nanosheets, the electrocatalytic performance might be impeded due to the inherent low electronic conductivity of Co3O4, as reported previously[117,118]. Carbon materials with high electronic conductivity promote efficient electron transfer from the electrode material to the reaction interface. Therefore, as shown in Figure 6A, Li et al. reported a thin mesoporous nanosheet Co3O4/nitrogen-doped reduced graphene oxide (Co3O4/N-rGO) as a bifunctional catalyst[119]. The hybrid ZAB exhibited superior discharge and charge polarization properties owing to the strong synergetic effect between the atomically thin Co3O4 and N-rGO. Doping is a similarly effective strategy that can further enhance the activity of TMOs[120-122]. For instance, Li et al. synthesized Ni-doped CoO nanosheets (Ni-doped CoO NSs) through a straightforward cation exchange process, employing ZnO nanosheets as sacrificial templates[43]. They demonstrated that uniform Ni doping effectively modulates the binding energy of the intermediate species in the ORR process, thereby enhancing the intrinsic ORR activity at each active site [Figure 6B][43]. The Ni-doped CoO NSs were integrated into ZABs, resulting in a high peak power density of 377 mW cm-2 and long-term stability for more than 400 h under a current density of 5 mA cm-2. Li et al. successfully obtained a high-performance bifunctional catalyst through doping Cu, N, and P into the precursor of the cobalt manganese oxides nanosheets/CNTs (N/PCu0.1Co0.3Mn0.6O2/CNTs)[44]. The effective modulation of the Cu, P, and N atoms on the number and the intrinsic activity of the active Co catalytic sites meant that the as-formed N/PCu0.1Co0.3Mn0.6O2/CNTs functioned as a bifunctional catalyst in ZABs. The creation of oxygen vacancies emerges as an appealing approach to modify the surface chemistry of TMOs, thereby enhancing their electrocatalytic performance[123-125]. Oxygen vacancies lead to an augmentation in electron density within neighboring metal atoms, thereby improving the conductivity of TMOs and facilitating charge transfer during the ORR and OER[126,127]. Tian et al. successfully synthesized nanosheets of oxygen-defective amorphous-crystalline CoO (ODAC-CoO) by fast calcination of Co(OH)2 under vacuum conditions[45]. The abundant active sites offered by its 2D structure and the incorporation of the amorphous phase led to an amorphous structure that effectively lowered the energy required to form oxygen vacancies, facilitating the creation and stabilization of these vacancies. Most importantly, the moderate oxygen vacancy content observed in ODAC-CoO-30 resulted in enhanced electrical conductivity and diminished energy barriers during ORR and OER processes. Consequently, the ODAC-CoO-30-based ZAB delivered a high peak power density of 128.5 mW cm-2 and a specific capacity of 705.6 mAh g-1. Except for the usual approaches to improve the catalytic properties of materials, the interfaces also play a pivotal role in enhancing the electrocatalytic properties of a nanocomposite catalyst, and both the heterojunction and fine-tuning of the electronic structure of the interface can be exploited. Liu et al. designed a mesoporous Ni/NiO (Porous Ni/NiO) nanosheet, and the assembled ZAB showed a high power density of 225 mW cm-2 and long-term cycling stability of over 120 h[46]. In addition, other heterojunctions such as Ru-RuO2[128], Cu/Fe2O3[129], and Fe2O3/Fe5C2/Fe-N-C[130] also show excellent activity in ZABs.
Figure 6. (A) (a) TEM image of the hybrid nanosheets; (b) ORR and OER polarization curves; (c) Discharge and charge polarization curves of the ZABs[119]. Copyright 2018, Wiley-VCH. (B) (a) SEM image of CoO NSs; (b) ORR polarization curves of pristine CoO NSs, CoO NSs with different Ni dopant concentrations and Pt/C; (c) Polarization curves and corresponding power density plots[43]. Copyright 2018, Wiley-VCH. (C) (a) TEM image of NiCo2.148O4 PNSs; (b) ORR polarization curves of Pt/C, Ir/C, NiCo2.148O4,
Considering that the introduction of additional metal ions can lead to the creation of more active sites, polymetallic oxides are anticipated to exhibit greater intrinsic oxygen electrochemistry activity as compared to single metal oxides[131]. Furthermore, the activity of polymetallic oxides can be readily enhanced by adjusting the respective metallic elements' crystalline structure, valence, and electronic states[132]. Typical spinel polymetallic oxides in the form AxB3-xO4 mainly include CoMn2O4, NiCo2O4, FeCo2O4, ZnCo2O4, and ZnMn2O4[133]. Of these, NiCo2O4, a representative spinel-structured oxide, continues to present limitations for application in ZABs, with many researchers attempting to improve its electrocatalytic performance.
TMOs, particularly those with perovskite structures, have been extensively explored as bifunctional electrocatalysts in ZABs due to their affordability, diverse compositions, structural stability, and promising potential[136-138]. However, pristine perovskite materials are still limited by their small active surfaces and fewer active sites. To further enhance their electrocatalytic activities, one effective strategy that can be used to promote electrocatalytic activity involves the introduction of other materials. Mondal et al. constructed the heterostructure of 2D Pr0.5Ba0.5Mn1.8-XNbXCo0.2O6-δ double perovskite oxide nanosheet (PBMNC) and NiFe LDH for ZABs[50]. The resulting PBMNC/LDH-20 cathode exhibited a high-power density of
LDHs possess a distinctive layered structure that consists of positively charged layers with negatively charged anions in the interlayer region. However, the practical utilization of LDHs in rechargeable ZABs is hindered by their limited ORR catalytic activity and low conductivity. Thus, Hui et al. designed MXene/Zn-LDH-array@PVA composite for use in ZABs[139]. The NiFe LDH with the best activity was modified by chemical induction to produce oxygen vacancies. The integrated interfacial structure of the MXene/Zn electrode and LDH-array@PVA electrolyte ensures sufficient interfacial contact with good compatibility and stability, reducing the interfacial impedance and limiting zinc dendrite growth. The assembled ZAB exhibited a promising peak power density (92.3 mW cm-2), and only a negligible voltage loss was found after continuous operation for 50 h. Oxygen vacancies modify the structure of active materials on an electrode/electrolyte interface without sacrificing the crystal stability[140,141], and experimental and DFT calculations have demonstrated that oxygen vacancies enhance the activity of a material for oxygen reactions[142]. Furthermore, for atomic adjustment, the core principle is optimizing the electronic structure at the active site[143,144]. For example, Han et al. configured atomic-level sulfur-incorporated NiFe-LDHs that were deposited in situ on nitrogen-doped graphene (S-LDH/NG) [Figure 6D][51]; the atomic-level sulfur incorporation resulted in a customized topological microstructure and adjusted electronic structure, leading to enhanced catalytic activity and durability of bifunctional electrocatalyst. Moreover, the theoretical calculations revealed that adjusting the electronic structure will impact the conductivity and increase the density of carriers, thereby significantly reducing the Fe-S site reaction energy barrier of zigzag edge structures. Consequently, S-LDH/NG-based ZABs demonstrated a high peak power density of 165 mW cm-2 and an energy density of 772 Wh kgZn-1.
Other 2D transition metal compounds
The distinct interlayer structure of 2D materials, such as TMSs, TMNs, and TMPs, have good electrical conductivity, corrosion resistance, and platinum-like electronic structures, highly desired properties for rechargeable ZABs. However, despite their excellent physical and chemical properties, they are prone to suffer from aggregation during synthesis, corrosion of catalyst supports, and catalytic instability during OER/ORR processes. To address these concerns, various methods have been explored to enhance their performance, such as regulation of reaction surface area, intrinsic catalytic activity, and conductivity[89,145,146].
During charging and discharging, the ORR and OER processes occur at the electrocatalyst's surface, giving rise to a triple interface region. To enhance the reactive surface area, many structures have been developed to promote the exposure of active sites. For instance, Cheng et al. synthesized carbon cloth-supported cobalt phosphide (CoP@CC) by in situ thermal phosphorylation[52]. As illustrated in Figure 7A, the crisscrossing sheets promote mass transfer between reactants and intermediates, thus avoiding aggregation of the CoP nanosheets that provide the active sites. Notably, the ZAB assembled with CoP@CC catalyst delivered a superior OCV of 0.6 V and a power density of 30 mW cm-2. Compared with CNTs and carbon nanofibers (CNFs), graphene, the classic 2D material, possesses a higher specific surface area and better stability and is an ideal substrate for new high-performance bifunctional catalysts. Ultrathin 2D NiCo2S4 nanosheets with a reduced graphene oxide hybrid material (NiCo2S4/rGO) structure possessed good electrocatalytic activity for OER and ORR[147]. The hybrid catalyst shows a unique hierarchical structure with a large surface area. The ZAB of NiCo2S4/rGO showed a high-power density of 262.6 mW cm-2 and remains stable even after
Figure 7. (A) (a) SEM image of Co(OH)2@CC; (b) ORR performance of CoP and commercial Pt/C; (c) Discharge polarization curve and corresponding power density in ZABs[52]. Copyright 2017, the Royal Society of Chemistry. (B) (a) SEM image of Co3FeN nanosheets; (b) LSV curves of different samples for ORR; (c) Discharge polarization curves and corresponding power density curves of different samples[54]. Copyright 2019, the Royal Society of Chemistry. (C) (a) SEM image of Re-Ni3S2/NG/NF; (b) ORR polarization curves of samples; (c) Charge/discharge polarization curves and power density plots Re-Ni3S2/NG/NF and Pt/C + IrO2/NF based ZABs[55]. Copyright 2022, Elsevier. (D) (a) SEM image of Pt/NBF-ReS2/Mo2CTx; (b) ORR and OER polarization curves of the different samples; (c) Discharge polarization curves and related power density plots in ZABs[57]. Copyright 2021, Elsevier.
Apart from maximizing the active surface area, effective manipulation of the intrinsic properties of active sites is crucial to enhance electrocatalyst performance. The introduction of heteroatoms is a widely used strategy to modulate atomic and electronic structures, which can affect the adsorption energy. As shown in Figure 7C, Han et al. reported a novel Re-doped Ni3S2 nanosheet array on N-doped graphene-modified nickel foam substrate (Re-Ni3S2/NG/NF) through via a simple hydrothermal strategy[55]. The unique structure endows Re-Ni3S2/NG/NF with a large active surface area and numerous electroactive sites. The incorporation of Re modulated the electronic structural states of Ni3S2, leading to a significant enhancement in electrocatalytic performance. As a result, the Re-Ni3S2/NG/NF-based ZAB exhibited a high-power density of 99 mW cm-2 and can be operated for 5,000 cycles. Similarly, Xu et al. designed Co atom-doped tungsten sulfides (Co-WS2) via a filtration and annealing process in the H2 atmosphere[148]. Co doping effectively regulates the electron structure of WS2, significantly boosting the ORR and OER performance, and the assembled rechargeable ZABs produced by Co-WS2 exhibited a high-power density of 211 mW cm-2. Two-dimensional materials offer a convenient platform for the modulation of surface/interfaces, resulting in enhanced OER and unleashed ORR activities. Robust interface interactions, unsaturated coordination, and doping with 2D materials can substantially alter the catalyst's electronic structure, thereby driving improvements in electrocatalytic performance. A rechargeable aqueous ZAB using the 2D NiFeMn nitride stabilized by Ti3C2 sheets as an air cathode endowed with a high specific capacity of 630 mAh gZn-1 and excellent cycling stability over 120 cycles[149]. This is attributed to enhanced active site exposure, charge redistribution, and the emergence of new active sites at the M-N-C or M-N-e interfaces, contributing to the notable OER activity and unlocked ORR reactivity.
Given that catalytic reactions transpire at the surface/interface of catalysts and inherently involve charge transfer, achieving swift and efficient charge transfer can mitigate polarization effects and expedite kinetic processes[150,151]. For instance, ultrathin oxygen-doped FePSe3 (FePSe3-O) nanosheets exhibit notable reorganization of surface atoms, resulting in the formation of multiple crystalline-amorphous interfaces that enhance the kinetics of the OER[56]. DFT calculations suggest that oxygen doping can also influence the electronic states at the Fermi level, leading to a reduced band gap that contributes to improved electrocatalytic performance. Moreover, 2D Co/Ce bimetallic nitrogen-doped carbon nanosheets
The discovery of 2D MXenes dates back to 2011[153]. The hydrophilic surfaces and high metallic conductivities of MXenes have led to their extensive utilization in various applications such as photodetectors[154,155], metal-ion batteries[156], metal-sulfur batteries, supercapacitors, and water splitting[157,158]. As emerging 2D nanosheets, MXenes have received extensive attention and are widely applied as effective catalysts in ZABs. For example, Chen et al. designed a composite by combining exfoliated V2C nanosheets with FeNi-LDH nanosheets, wherein the hypophosphite groups were introduced as intercalated anions in the LDHs layers (referred to as H2PO2-/FeNi-LDH-V2C)[159]. The strong interfacial interaction and electronic coupling between the LDHs and V2C nanosheets guarantee fast charge transfer kinetics and a stable structure. The assembled ZAB of H2PO2-/FeNi-LDH-V2C showed a superior OCV of 1.42 V, a high-power density (137 mW cm-2), and long durability. In others, Zou et al. proposed a novel hierarchical porous Ni-Co-mixed metal sulfide (NiCoS) on Ti3C2Tx MXene via a MOF-based approach[160]. Taking advantage of the unique structure and strong interfacial interaction of NiCoS and Ti3C2Tx, the hybrid material demonstrates notable electrical conductivity, facilitating efficient electron transport. Consequently, the assembled ZABs that utilize the NiCoS/Ti3C2Tx material exhibit a small charging/discharging voltage gap and excellent durability for long-term operation. MXenes are usually used in forming composite materials with other compounds or SAs. Yi et al. synthesized N, B, and F atom-doped ReS2, which is grown evenly on the surface of MoC2Tx MXene, followed by a uniform loading of single-atom Pt on NBF-ReS2 (Pt/NBF-ReS2/Mo2CTx) electrocatalysts, and utilizing the 2D morphology of ReS2 to offer an extensive surface area for the incorporation of individual Pt atoms[57], as seen in Figure 7D. In addition, the introduction of N, B, and F increases the number of active sites. The assembled ZAB with the Pt/NBF-ReS2/Mo2CTx electrode exhibited a narrow charge-discharge gap, a higher peak power density of
Others 2D catalysts
Apart from the aforementioned 2D nanomaterials, other 2D materials, such as MOFs, COFs, C3N4, and BP, have also been applied in the development of ZABs. For example, 2D MOFs can be synthesized from the strong coordination covalent bonds that form between metal-containing nodes and active organic components and can function as the active air cathode in aqueous ZABs. Recently, Jiang et al. incorporated ligand vacancies and hierarchical pores into cobalt-zinc heterometal imidazole frameworks (BHZ-48) to regulate their electronic states and structural porosity [Figure 8A][58]. In the synthesized BHZ-48, the orbital configuration of active sites is influenced by both near-range interactions with ligand vacancies and distant interactions with the Co-Zn alliance. This combination results in optimized adsorption/desorption energy for the reactive intermediates, effectively lowering the energy barrier for the potential-determining step. When employed as an air electrode, the BHZ-48-based ZAB exhibited a high-power density (148 mW cm-2 at 250 mA cm-2) and excellent durability (1,250 h). In addition, because a single catalyst alone cannot achieve optimal activities for ORR and OER, it is essential to devise strategies that effectively balance the absorption and desorption energies of oxygen intermediates in bifunctional catalysts so that ORR and OER can be facilitated simultaneously. Jiang et al. alter the intermediate adsorption free energy on the Co-Fe zeolitic imidazolate framework (CFZ)[8]. Both the experimental results and DFT calculations demonstrate that the electron-withdrawing characteristic of the sulfur group finely tunes the energy level of the d-orbital in the cobalt, regulating the free energy of OH* adsorption and desorption (ΔGOH*) and leading to exceptional catalytic activity. The assembled ZAB exhibits remarkable long-term durability of 525 h.
Figure 8. (A) (a) SEM image of BHZ-48; (b) ORR LSV curves for comparison of different samples; (c) Charge and discharge polarization curves[58]. Copyright 2020, Springer Nature. (B) (a) SEM image of FeNi LDH-TpF6-2; (b) FT-IR spectra; (c) Discharge and charge polarization and power density curves[59]. Copyright 2023, Wiley-VCH. (C) (a) SEM image of Co-CNNs-0.7; (b) ORR polarization curves for various catalysts; (c) Polarization and power density curves[60]. Copyright 2020, the Royal Society of Chemistry. (D) (a) TEM image of BP-CN-c; (b) P L2,3-edge XANES spectra of BP, BP-CN-p, and BP-CN-c; (c) Discharge/charge polarization curves of ZABs assemble with BP-CN-c and Pt/C+RuO2[62]. Copyright 2021, Wiley-VCH.
In addition to the electronic structure of the MOF, the morphology of the utilized material has been identified as a critical factor in enhancing catalytic activity. Franco et al. synthesized an ultrathin 2D Cu-based MOF (2DCIF) that demonstrated remarkably high ORR activity[161]. Moreover, the presence of uniformly distributed Cu-N4O units within a stable 2D framework allows successful utilization of the 2D coordination-included frameworks as air electrodes in aqueous ZABs with outstanding specific capacity and power density values. This success underscores the excellent attributes of 2D Cu-based MOFs as electrocatalysts for energy storage devices. Additionally, integrating specific conductive substrates with MOFs has been explored to effectively enhance the electron migration, further augmenting the ZABs properties. In a representative study, a composite was fabricated by Zhao et al. by in situ hybridizing stable, hydrophilic, and conductive MXene nanosheets with 2D cobalt 1,4-benzenedicarboxylate
Two-dimensional COFs are constructed using symmetric organic building blocks and are self-assembled through π-π stacking interactions. Their structural adjustability, finely tuned porosity, and strong chemical stability render them promising catalysts for application in aqueous ZABs[164,165]. Cao et al. designed a novel fluorinated COF engineering strategy to develop a high-performance catalyst for rechargeable ZABs[59]. As shown in Figure 8B, fluorinated COF (TpFx-COF) in situ was grown on the hydrophilic FeNi LDH to obtain the FeNi LDH-TpFx and showed high O2 affinity and several nanopores. The TpFx-COF with well-defined channels can act as the aerophilic “nano-islands” for the transportation of O2 on the FeNi-LDH electrocatalyst surface. This arrangement effectively segregates the mass O2 and water transport pathways on the nanoscale, substantially increasing the three-phase boundary area and significantly enhancing the mass transfer efficiency. The assembled ZAB of TpFx-COF exhibited a narrow charge/discharge voltage gap of 0.64 V, a peak power density of 118 mW cm-2, and stable cyclability.
A 2D material that is composed of periodic aromatic units consisting of sp2-hybridized carbon and nitrogen atoms, g-C3N4, is characterized by a low surface area and inadequate conductivity, which hampers its viability as an effective electrocatalyst for ORR and OER. Therefore, the design of a g-C3N4-based electrocatalyst with high electron mobility for the efficient catalysis of ORR and OER in ZABs remains unfulfilled[166,167]. Recently, Niu et al. synthesized graphitic carbon nitride embedded with TM nanocrystals (M-CNN) through a general solid-phase pyrolysis method [Figure 8C][60]. The coordination bond between the metallic Fe, Co, Ni, and N in the M-CNNs-x composites resulted in more defective and disordered graphitic structures than CNNs. Moreover, the ZAB with the Co-CNNs-0.7 composite as an air cathode demonstrated a notable peak power density of 85.3 mW cm-2 and a specific capacity of 675.7 mA h g-1. Since then, other g-C3N4-based composite catalysts with abundant M-Nx active sites, such as Fe-NC&CN[168], NGM-CN-Fe[169], and Co-NC-900[61], have been developed. The assembled ZABs that use these materials exhibit high specific capacity with stable and persistent discharge performances.
BP was first synthesized in 1914; however, it was only in 2014 that theory and experimentation confirmed the presence of a BP monolayer. This revelation piqued the interest of researchers[170], and 2D BP as a multifunctional material, which has many merits, including the adjustable bandgap, puckered-honeycomb configuration, and high charge carrier mobility, attracted much attention in the energy storage field[171-173]. However, challenges such as low catalytic activity and inadequate electrochemical stability currently limit its application. Because of this, Wang et al. developed a metal-free bifunctional oxygen electrocatalyst based on BP and g-C3N4, denoted as BP-CN-c, in which BP nanosheets were covalently bonded to g-C3N4. This material was produced by mixing BP nanosheets with g-C3N4 and subsequently subjecting the mixture to anneal at 300 °C under a nitrogen atmosphere, as shown in Figure 8D[62]. The polarized P-N covalent bonds in BP-CN-c effectively modulate the electron transfer from BP to graphitic carbon nitride, markedly enhancing the adsorption of OOH* onto phosphorus atoms. Consequently, the ZAB assembled by BP-CN-c cathode exhibited a high-power density of 168.3 mW cm-2 and an energy density of 952.7 Wh kg-1.
2D MATERIALS FOR NONAQUEOUS LOBs
Despite both falling under the category of MABs, the reaction mechanisms of LOBs and ZABs significantly differ due to variations in the electrolyte composition. In ZABs, the reduction of oxygen to hydroxide during discharge and the oxidation of water to release oxygen during charging are the most critical reactions, and their slow kinetics restrict the development of batteries. However, to LOBs, the development is mainly subject to the generation and decomposition of Li2O2, which affects round-trip efficiency, degraded capacity, and cyclability. In response, substantial research endeavors have been directed towards the exploration and application of efficient 2D materials as potent electrocatalysts in response to these challenges. In this context, diverse 2D catalysts, including carbon-based materials, metal-decorated 2D carbon structures, TMOs/TMOHs, other TM compounds, C3N4-based materials, and 2D MOF/COFs, have been employed in LOBs, yielding varying degrees of success. The detailed electrochemical performances of these materials as catalysts in LOBs are listed in Table 2.
Comparison of different types of 2D electrocatalyst performances in LOBs
Catalysts | Electrolyte | Discharge specific capacity | Current density (mA g-1) | Limited capacity@current density | Cycle number | Refs. | |
2D Carbon-based materials | NS-rGO | 1 M LiTFSI/TEGDME | 3,320 | 0.24 | 1,000@ 0.24 mA cm-2 | 14 | [174] |
NS-G-700 | 1 M LiTFSI/TEGDME | 1,000 | 100 | 1,000@600 | 25 | [175] | |
s-BNC | 1 M LiTFSI/TEGDME | 8,397 | 1,500 | 1,000@500 | 160 | [176] | |
S-doped graphene | 1 M LiTFSI/TEGDME | 10,400 | 200 | 1,000@300 | 100 | ||
B-hG-700 | 1 M LiTFSI/TEGDME | 19,698 | 100 | 1,000@100 | 120 | [178] | |
GDY/Fc | 1 M LiTFSI/TEGDME | 14,231 | 200 | 500@200 | 183 | [13] | |
Metal-decorated 2D carbon materials | Ru/N-rGO | 1 M LiTFSI/TEGDME | 17,074 | 500 | 1,000@100 | 100 | |
Pt-HGNs | 1 M LiTFSI/TEGDME | 5,600 | 100 | 1,000@100 | 54 | [180] | |
Ir-rGO | 1 M LiTFSI/TEGDME | 9,500 | 100 | 1,000@100 | 40 | ||
CoCu/graphene | 1 M LiTFSI/TEGDME | 14,821 | 200 | 1,000@500 | 122 | [182] | |
PVP-C@LDO | 1 M LiTFSI/TEGDME | 4,333 | 100 | 1.3 mAh cm-2@100 | 70 | ||
Co-SAs/N-C200 | 1 M LiTFSI/TEGDME | 11,098 | 1,000 | 1,000@200 | 260 | ||
Co-SA-rGO | 1 M LiTFSI/TEGDME | 12,760 | 100 | 1,000@100 | 220 | ||
RuNC/CoSA-3DNG | 1 M LiTFSI/TEGDME | 25,632 | 100 | 1,000@200 | 300 | ||
2D transition metal oxide/hydroxide | 1% Ir1/Co3O4 | 1 M LiTFSI/TEGDME | 16,861 | 200 | 500@200 | 180 | |
CoFeCe-2 oxide | 1 M LiTFSI/TEGDME | 12,340 | 100 | 1,000@100 | 2,900 h at 100 mA g-1 | ||
SeZnO3 nanosheets | 1 M LiTFSI/DMSO | 13,200 | 500 | 1,000@500 | 140 | ||
LaFeO3-x NS | 1 M LiTFSI/TEGDME | 14,983 | 100 | 1,000@100 | 60 | [190] | |
Other 2D transition metal compounds | NiS2-NSs | 1.0 M LiNO3 in DMA | 22,500 | 1,000 | 1,000@1,000 | 300 | |
Co3O4-NS | 1 M LiTFSI in TEGDME | 5,917 | 100 | 500@100 | 25 | [192] | |
H-2D-Co9S8 | 1 M LiTFSI in TEGDME | 3,500 | 100 | 500@100 | 100 | ||
NiS2-CoS2@NC | 1 M LiTFSI/TEGDME | 14,551 | 1,000 | 500@500 | 490 | ||
CuCo2S4@Ni | 1 M LiTFSI/TEGDME | 9,763 | 100 | 500@200 | 164 | ||
2D Bi2Te3 | 1 M LiNO3/DMSO | 21,172 | 500 | 600@500 | 188 | [196] | |
MoS2/AuNPs | 1 M LiTFSI/TEGDME | 3,567 | 600 | - | 50 | ||
MoS2-x@CNTs | 1 M LiTFSI/TEGDME | 19,989 | 200 | 1,000@200 | 105 | ||
Ni/Mn-MoS2 | 1 M LiTFSI/DMSO | 28,195 | 100 | 1,000@200 | 118 | ||
Pd-TMS/CT | 1 M LiTFSI/TEGDME | 7,441 | 500 | 1,000@500 | 622 | ||
6Ag-SnSe2 | 1 M LiTFSI/DMSO | 16,871 | 100 | 1,000@1,000 | 144 | ||
Co2P/acetylene black | 1 M LiTFSI/DMSO | 5,102 | 0.1 mA cm-2 | 500@0.1 mA cm-2 | 132 | ||
N-TiO2/Ti3C2Tx | 1 M LiTFSI/TEGDME | 15,298 | 100 | 500@500 | 200 | ||
Other 2D catalysts | 2D Mn-MOF | 1 M LiTFSI/TEGDME | 9,464 | 100 | 1,000@100 | 200 | |
Co-MOF/MXene | 1 M LiTFSI/TEGDME | 34,763 | 1000 | 1,000@1,000 | 278 | ||
CMT@MXene | 1 M LiTFSI/TEGDME | 6,850 | 200 | 1,000@500 | 247 | ||
Ni-TAPP-Co | 1 M LiTFSI/TEGDME | 17,104 | 500 | 1,000@200 | 200 | ||
Pt-CNHS | 1 M LiTFSI/TEGDME | 17,059.5 | 100 | 600@100 | 70 | ||
Ag/g-C3N4/ | 1 M LiTFSI/TEGDME | 7,723 | 100 | 1,000@100 | 51 |
2D carbon-based materials
Since the first application of graphene nanosheets[210], various 2D carbon materials have been employed as cathodes in LOBs. These 2D carbon materials mainly include graphene, precursor-derived carbon, and graphylene[13,211,212]. This choice is not only driven by the cost-effectiveness, abundant availability, and low mass density of carbon-based 2D materials but is also due to their inherent ORR activity. However, it is important to acknowledge that the further development of these 2D carbonaceous materials still faces challenges related to their architectural design. Without advanced architecture, the smooth promotion of mass transfer for all reactants becomes compromised, and chemical pathways are susceptible to blockage due to the deposition of insoluble discharge products.
To address these challenges, substantial endeavors have been directed towards the development of porous carbon materials with advanced structures. Broadly speaking, these approaches fall into two broad categories, the first of which involves manipulating the in-plane structure of monolayer graphene. Lin et al. achieved holey graphene via facile air oxidation methods and further designed air electrodes with porous structures[213]. When employed in LOBs, the high mass loading air cathode exhibits ultrahigh areal capacity reaching approximately 40 mA cm-2. Another technique involves adjusting the hierarchical structure of 2D nanosheets. Jung et al. developed a free-standing film with robust geometric characteristics by non-stacked graphene that could function as a cathode in LOBs[174]. The considerable porosity confirmed by Brunauer-Emmett-Teller (BET) surface area measurement led to significantly improved electrochemical performance in terms of discharge capacity. In light of the aforementioned findings, 2D carbon materials with advanced architecture inherently provide abundant voids and spaces that facilitate oxygen-related reactions, ensure convenient access to the electrolyte, and enable swift oxygen diffusion. These effects hold the potential to further elevate the overall electrochemical efficiency of LOBs.
However, the intrinsic limitations of pure 2D carbonaceous materials in effectively catalyzing the formation and decomposition of Li2O2 have constrained their application within LOBs. The low kinetic and thermodynamic activity of those untreated carbon-based catalysts will lead to a large overpotential and induce an irreversible capacity fading, which may lead to the battery failure. Also, the common carbon electrode in LOBs still suffers from the inevitable side effects, such as the nucleophilic attack with Li2O2, further forming Li2CO3. Fortunately, the incorporation of heteroatoms, such as N, P, B, O, and S, presents a viable solution to improve the surface electronic structure of pristine materials and establish new active sites[175,176,178,211,212,214]. This approach offers a promising opportunity to boost the ORR/OER catalytic performance of carbon materials. Also, it is demonstrated to mitigate the side effects. In the case of N doping, the presence of electron-accepting N atoms introduces a higher positive charge density to the neighboring carbon atoms. Computational and experimental studies have indicated that introducing nitrogen atoms enhances the electrocatalytic activity of nanostructured carbon materials toward oxygen reduction[77]. Distinct from N atom doping, doping graphene with B not only expedites the kinetics of the ORR but also affects the decomposition of Li2O2. According to first-principle thermodynamics calculations, B-doped graphene can reduce the rate-determining barrier to further improve the charge rate of LOBs[215]. Furthermore, recent investigations have demonstrated that the simultaneous incorporation of multiple heteroatoms into graphene yields hybrid structures with supplementary catalytic properties attributed to their synergistic coupling effects[175,176].
Based on the research findings presented, it becomes evident that combining porous 2D carbon materials with hierarchical structures and heteroatom-doping holds significant promise and may potentially pave the way for the development of advanced LOBs. For example, Han et al. demonstrated that the synergistic effect of chemical doping and 3D porous configuration substantially improved the reaction kinetics of electrodes[177]. Nitrogen and sulfur-doped nanoporous graphene, while retaining its lightweight nature, ample accessible surface area, and high conductivity, exhibited substantial capacity and excellent cycling stability in rechargeable LOBs. In another study, Liu et al. designed an edge-rich porous carbon structure that resembled stacks of holey graphene via COF pyrolysis, which was denoted COF800 [Figure 9A][212]. Heating led to the decomposition of nitrogen-containing groups within the COF to produce graphitic and pyridinic nitrogen species. The uniform nitrogen doping and edge sites in the endowed COF800 resulted in heightened intrinsic catalytic activity toward ORR. Meanwhile, the COF800-based LOB also exhibited a high discharge specific capacity of 2,558 mAh g-1. Similarly, boron-doped holey graphene (B-hG-700), with a significant number of holes and a high boron doping level of 6 at.%, was reported to exhibit exceptional performance in LOB including a high capacity of 19,698 mA h g-1 at 100 mA g-1, and remarkable cycling stability over 120 cycles [Figure 9B][178]. Both experimentation and DFT calculations indicated a preference for Li2O2 nucleation and vertical growth near the hole/edge sites, facilitating isolated and uniformly distributed Li2O2. This process effectively reduces Li2O2 agglomeration and creates abundant space to accommodate Li2O2, thereby enhancing oxygen reduction and evolution kinetics.
Figure 9. (A) (a) TEM images of COF800; (b) Pore size distribution curves of the COF and COF treated at different temperatures; (c) Initial GCD profiles of LOBs based on COF800 and Ketjen Black at 0.05 mA cm-2[212]. Copyright 2021, Wiley-VCH. (B) (a) TEM image of B-hG-700; (b) Nitrogen adsorption-desorption curves of samples; (c) Initial discharge-charge curves of different cathodes at
Besides, 2D carbon materials can also be employed to mitigate severe redox shuttling. A novel carbon family, namely the GDY matrix, was introduced by Li et al. for this purpose [Figure 9C][13]. The GDY framework anchored with ferrocene (GDY/Fc) serves as a stationary soluble redox mediator that preserves the redox-mediating ability of ferrocene while promoting the oriented 3D growth of Li2O2, effectively mitigating the shuttle effects that are associated with the redox process. LOBs based on the GDY/Fc electrode demonstrated a high discharge specific capacity of 14,231 mA h g-1 and long-term cycle stability of more than 183 cycles.
Metal-decorated 2D carbon materials
Even if element doping and porous structure construction strategies are used to improve the properties of 2D carbon materials, they are still insufficient to promote the ORR and OER kinetics in LOBs, which may result in high overpotential, low reversibility and poor rate capability. To address these challenges, the incorporation of metals with varying sizes into 2D-based carbon materials has been pursued. This approach can be categorized into three types: ultrasmall nanoparticles, SAs, and combinations of ultrasmall nanoparticles with SAs.
Considering the challenge of dispersing metal nanoparticles, 2D carbon materials are highly suitable as carriers for precious metals due to their abundant active sites, which facilitate the anchoring and growth of precious metals. Sabatier's principle suggests that noble metal catalysts (Ru, Ir, Pt, and Pd) are ideal for promoting the O2/Li2O2 redox reaction in MABs due to their moderate binding energy with LiO2 intermediates[216]. Therefore, tremendous noble metal nanoparticles anchored on functionalized carbon nanosheet catalysts have been designed[179-181,217,218]. Among them, crystalline Pd, situated near the apex of the "volcano plot," demonstrates optimal interactions with LiO2 for ORR in LOBs. Both flexible Pd nanodendrites on graphene nanoplatelet[219] and Pd nanoparticles coordinated with rGO[217] exhibited significantly reduced overpotentials in nonaqueous LOBs. Similarly, Ru possesses strong adsorption energy for LiO2 intermediates and effectively promotes the decomposition of discharge and side products, aligning with the design principles for efficient Li-O2 catalysts. Dai et al. designed N-doped reduced graphene oxide anchored by ultra-dispersed Ru nanoparticles for LOBs[179]. The Ru active centers and strong metal-support interactions were found to facilitate a viable process of forming and decomposing discharge products by influencing the surface adsorption of lithium superoxide intermediates and the nucleation and growth of lithium peroxide species. According to these advantages, this electrode exhibited better performance in the LOB, including enhanced discharge capacity of 17,074 mA h g-1, decreased charge overpotential, and long-term stability. Other precious metal-2D carbon materials are also emerging in the field of LOBs. With a strong oxygen absorption ability, Pt nanoparticles with ultrasmall sizes coated onto hollow graphene nanocages act as catalytic nucleation sites of Li2O2[180]. The distinctive matrix of hollow graphene nanocages serves a dual purpose: it not only furnishes a plethora of nanoscale tri-phase regions, serving as active sites for efficient oxygen reduction, but also provides an ample quantity of mesoscale pores, facilitating rapid oxygen diffusion. The catalysts finally lead to the subsequent formation of Li2O2 in a favorable form characterized by small size and an amorphous state, facilitating more efficient decomposition. Iridium incorporated into deoxygenated hierarchical graphene[220] and Ir-rGO composites[181] are also demonstrated as the advanced catalysts to accelerate the formation of Li-based intermediate and oxygen evolution. Nevertheless, the cost and scarcity of these metals limit their use as catalysts in commercial LOBs and have led to the exploration of non-precious metal nanoparticles on 2D carbon substrates, particularly cobalt-based catalysts. The 3D metal of Co, strongly bonded to LiO2, has demonstrated superior catalytic activity toward Li2O2 formation and decomposition[182,183]. In addition, it was demonstrated that bimetallic catalysts comprising two distinct metals may show exceptional catalytic activity, leveraging the synergy between the metals. Cobalt-copper bimetallic nanoparticles supported on graphene as cathode materials in LOBs exhibited superior rate capability, long cyclability, and outstanding coulombic efficiency due to the synergy of Co and Cu on graphene, enhancing both oxygen reduction and evolution kinetics[182]. Co and CoFe alloy nanoparticles decorated 2D NC also exhibited outstanding ORR/OER performances and durability in LOBs[183].
Compared to solid nanoparticles, SAs have garnered considerable attention in catalytic systems owing to their exceptional properties, which encompass abundant non-saturated atomic coordination sites, efficient utilization of active atoms, and uniform active centers. Notably, in LOBs, single metal active sites are beginning to exhibit substantial potential in accelerating reaction kinetics and enhancing active species utilization effectively. An exemplary instance involves the synthesis of ultrathin nitrogen-rich carbon with embedded isolated cobalt atoms (Co-SAs/N-C) as the catalyst for LOBs [Figure 10A][184]. The produced catalyst full of exposed atomic active sites demonstrates a favorable capacity to control the formation and decomposition of ultra-distributed Li2O2 during ORR and OER. These benefits stem from the abundant Co-N-C catalytic sites, leading to significantly enhanced kinetics and reduced overpotentials. Notably, theoretical simulations have revealed that the plentiful cobalt-nitrogen moieties can substantially increase the affinity of active species during reaction. This adjustment completely modulates the formation mechanism of the final product Li2O2 in terms of size and distribution. Accordingly, the LOB with Co-SAs/N-C cathodes achieved an excellent discharge capacity of 20,105 mAh g-1 and a long-term cyclability of 260 cycles. Recently, it has been found that LOBs with LiOH redox chemistry hold great promise for practical applications with an improved energy density and cyclic lifetime[221,222]. Also, LiOH, as a discharge product, exhibits greater chemical stability than Li2O2. Single-atom Co-N4/graphene catalysts (Co-SA-rGO) derived from MOFs were introduced and underwent 4e- catalytic reactions with the product LiOH while mitigating water shuttling[185]. The 3D networks of Co-SA-rGO deliver substantial surface area and mesoporous structures, facilitating both the capture of ≈12 wt% H2O molecules and rapid pathways for O2 diffusion and Li+ transportation. Additionally, the Co-N4 center demonstrates enhanced activity toward proton-coupled electron transfer, favoring the 4e- formation of LiOH. With these unique features, the LOB based on Co-SA-rGO achieves a high discharge voltage platform of 2.83 V and a substantial discharge capacity of
Figure 10. (A) (a) Zoom-in HAADF-STEM image of the Co-SA/NC catalyst; (b) K-edge FT-EXAFS in R space for related samples; (c) Initial deep GCD curves of related samples[184]. Copyright 2020, Springer Nature. (B) (a) HAADF-STEM images of RuNC/CoSA-3DNG; Fourier-transform EXAFS spectra for (b) Ru K-edge; (c) Initial discharge-charge profiles (voltage: 2.0-4.5 V, current density:
Through precise manipulation, both metal SAs and particles can coexist on the surface of 2D materials, showcasing broad prospects in the catalysis field. For instance, Ru nanoclusters were integrated onto N-doped 3D rGO alongside single Co atoms (RuNC/CoSA-3DNG), resulting in highly efficient cathode catalysts for LOBs [Figure 10B][186]. The catalyst exhibited outstanding performance, including low charge potential, high discharge capacity (25,632 mA h g-1), and superior cycling stability (300 cycles). The study revealed that the introduction of single Co atoms resulted in the charge transfer between Ru clusters and other atoms and finally led to the electron-deficient Ru. The unique chemical environment of Ru enabled effective adsorption and activation of negatively charged O22- ions in Li2O2, further improving the OER activity. Additionally, the well-dispersed Ru clusters facilitated the generation of Co-N-C with enhanced ORR and OER activities, thereby leading to excellent cathodic performance in LOBs.
2D transition metal oxide/hydroxide
According to the studies, both 2D noble metal oxides[223,224] and TMOs[16,225-230] with 2D morphology are promising candidates as catalysts in LOBs. However, a critical challenge lies in the scarcity of precious metal resources on our planet, which hinders its large-scale application in LOBs. The high cost of these materials, coupled with their susceptibility to degradation due to metal poisoning or leaching, further complicates the issue. Consequently, the subsequent discussion will concentrate on efficient TMO catalysts for LOBs. Of all TMOs, Co3O4 nanosheets in which Co2+ and Co3+ ions occupy the tetrahedral and octahedral sites have been most thoroughly studied in terms of geometry[231], crystal plane control[227,232], and crystal structures. With more research on SACs, Co3O4 nanosheets loaded with single-atomic metals (M-Co3O4) were devised and demonstrated as high-performance cathode catalysts [Figure 11A][187]. The incorporation of single-atomic metals can effectively replace the central Co atom in the octahedral coordination structure, maintaining its structural integrity. This alteration offers an electron promotion effect, thereby exposing more active Co3+ sites, which, in turn, provides more nucleation locations for Li2O2 deposition. Furthermore, the introduced metal atoms can spatially segregate the active Co3+ centers, thereby regulating Li2O2 dispersion to yield a sheet-like morphology that facilitates subsequent charge cycle decomposition. Meanwhile, MnO2[225],
Figure 11. (A) (a) SEM image of Ir/Co3O4; (b) Fourier-transform EXAFS spectra of R space; (c) First discharge/charge curves[187]. Copyright 2023, Wiley-VCH. (B) (a) SEM image of CoFeCe oxide; (b) HRTEM image of CoFeCe oxide; (c) Full first discharge/charge curves of as-prepared catalyst-based LOBs at 100 mA g-1 under a potential of 2.2-4.4 V (vs. Li+/Li)[188]. Copyright 2021, Wiley-VCH. (C) (a) SEM images of LaFeO3-x NS; The Fe L-edge (b) and O K-edge (c) The initial capacity of samples at the current density of
Perovskite materials possess adjustable physical and chemical properties and can catalyze both ORR and OER, rendering them promising and cost-effective alternatives to noble metals for cathode catalyst application. Guided by the design principle of an eg-filling with a value of ~1 for maximum ORR activity[239] and recognizing the inherent low conductivity of perovskite, a meso-LaSrMnO layer supported on graphene nanosheet was synthesized as a catalyst for LOBs. This well-designed system capitalizes on its structural elements: the interstitial space between nanosheets facilitates diffusion of O2 and Li+, the mesoporous structure provides an extensive surface area for electrolyte penetration and discharge product deposition, the perovskite catalyst phase reduces reactive overpotential, and the graphene component establishes a conductive pathway for electron transport. The introduction of oxygen defects has been considered a significant approach to enhancing the catalytic performance and electrical conductivity of 2D perovskites[189,240]. It has been demonstrated that oxygen vacancies in SeZnO3 not only increase the number of catalytically active sites but also enhance electrical conductivity, thereby further elevating the catalytic efficacy for LOBs[189]. LOBs employing oxygen vacancy-enriched LaFeO3-x nanosheets (LaFeO3-x NS) as catalysts exhibited remarkable attributes such as low overpotential, high specific capacity (14,983 mAh g-1), and prolonged cycle stability (60 cycles) [Figure 11C][190]. These advantages stem from the synergistic interplay of engineering of 2D nanosheets, oxygen defects, and modulation of Fe valence. The choice of metal oxides is mainly concentrated in the oxides of iron, cobalt, nickel, and manganese from Table 2; among these, Co3O4 has been intensively studied. It is widely known that the high performance with enhanced long-term stability is hard to obtain with monometallic oxide materials without any further modifications, including both the modification of geometric and atomic structures. In the pursuit of high-efficiency catalysts for LOBs, TMOHs also play a significant role. TMOHs, especially LDHs, have garnered substantial attention owing to their tunable chemical properties and distinct 2D structures. While the limited catalytic prowess of TMOHs has historically hindered advancements in electrochemical performance, various strategies have been employed to overcome this limitation. These include the creation of metal vacancies[241], manipulation of crystal phases[242], and integration with conductive substrates[243,244]. Furthermore, Lu et al. successfully synthesized materials with a superlattice structure that comprised CoNiFe LDH and RuO2.1 nanosheets, demonstrating exceptional performance in LOBs[245]. The CoNiFe LDH carrying a positive charge and the negatively charged RuO2.1 are intricately arranged at the molecular level, forming superlattice-like hybrids through electrostatic interactions under specific conditions. This observed performance can be attributed to the unique superlattice structure, which facilitates robust interfacial electronic coupling, improved electrical conductivity, and the mitigation of side reactions often observed with conventional carbon-based materials.
Other 2D transition metal compounds
In addition to metal oxides, other compounds within the oxygen group (such as metal sulfides[191-195,246-249], selenide[250], and telluride[196,251]) and the nitrogen group (including metal nitrides and phosphides[202,252,253]) exhibit remarkable catalytic properties in LOBs. Among the oxygen group compounds, metal sulfides are the most widely studied. Following the initial investigation of NiS as a novel cathode catalyst for LOBs, numerous other TMSs have been explored and reported[254]. Binary metal sulfides have emerged as notable candidates for energy conversion and storage applications due to their affordability, abundant availability, heightened electron conductivity, and enhanced electrochemical activities.
As an example, single-crystalline NiS2 nanosheets (NiS2-NSs) have been synthesized and demonstrated as efficient electrocatalysts for high-performance LOBs[191]. These single-crystalline NiS2-NSs can effectively facilitate the reversible L2O2 formation and decomposition, respectively. Furthermore, 2D Co3S4 with the nano-thin sheet-like structure was fabricated via the simple hydrothermal method[192]. The nanosheets with distinctive mesopores exhibited exceptional electrochemical performance in LOBs with a first discharge capacity of approximately 5,917 mAh g-1 and a high reversibility of 95.72%. Moreover, flower-like copper sulfide[246] and defect-rich holey Co9S8[193] also exhibited significant performance in controlling the deposition of nanoscale Li2O2. Following the d-band center theory, the elevation (or reduction) of the d-band position corresponds to a heightened (or diminished) occupancy of the anti-bonding state, thereby resulting in a more potent (or weakened) adsorption of intermediates due to the less filling of the adsorbate-metal anti-bonding states. Consequently, adjusting the adsorption capacity by manipulating the d-band center has emerged as an efficacious approach to bolstering the electrocatalytic performance. Li et al. proved that an isomorphic structure, as illustrated by the cobalt/nickel pyrite composite (NiS2-CoS2@NC), can effectively customize the d-band center, thereby enhancing the adsorption ability of intermediates in LOBs
Figure 12. (A) (a) SEM image of NiS2-CoS2@NC; (b) Initial discharge/charge curves; (c) Density of states with dotted lines identified as the d-band centers of different catalysts[194]. Copyright 2023, Wiley-VCH. (B) (a) SEM image of Ag-SnSe2; (b) Initial discharge/ charge profiles of different cathodes at 100 mA g-1 with a voltage range from 2.35 to 4.35 V; (c) Calculated energy diagram of Ag-(001) plane in ORR and OER processes[201]. Copyright 2022, Wiley-VCH. (C) (a) TEM image of N-TiO2/Ti3C2Tx; (b) Discharge-charge curves of the first cycle in various samples; (c) Gibbs free energy diagrams for N-TiO2/Ti3C2Tx[203]. Copyright 2022, the American Chemical Society.
TMDCs, a typical 2D material, are also widely used in LOBs. Although they are not widely used in ZABs, they are considered to be excellent catalysts for the formation and decomposition of L2O2 due to their different reaction mechanisms. In 2015, MoS2 nanosheets decorated with gold nanoparticles (MoS2/AuNPs) were initially introduced as promising cathode materials for LOBs[197]. Since then, research on TMDCs for LOBs has been ongoing, mainly focusing on various aspects, including their integration with other materials, defect engineering, doping strategies, and phase modulation. As a typical inorganic compound, conductivity is the key problem that hinders the application of TMDCs in the battery field. Therefore, conductive carriers are often used to construct complexes to improve this situation, such as core-shell nanostructured MoSe2@CNT[256], 2D MoS2 nanosheets anchored on hollow carbon spheres (MoS2/HCS) composites[257], and graphene-like MoSe2 nanosheets anchored on hollow CNFs[258]. As far as defects are concerned, the dominant focus is on the study of the non-metallic vacancy[198,259]. For example,
Metal phosphide, included in nitrogen group compounds, such as Co2P[202], Ni2P[252], and MoP[253], possess an intrinsic metallic nature, facilitating excellent electrical conductivity and rapid electron transfer at the electrode/electrolyte interface. These attributes position them as highly promising alternatives for oxygen electrode materials in LOBs. Huang et al. developed porous cobalt phosphide nanosheets with a high surface area using an environmentally benign hydrothermal approach[202]. The resulting Co2P/acetylene black composite exhibited improved electrochemical performances, featuring high capacities of
2D MXenes (TMCs/TMNs) have emerged as a fascinating candidate for electrodes in LOBs due to their remarkable attributes, such as high electrical conductivity, tunable layered structure, abundant active sites, and adjustable surface groups. Theoretical simulations have indicated that MXene phases, such as Ti3C2Tx, hold the potential for superior catalytic performance in LOBs by establishing stable and conductive two-phase interfaces with Li2O2. Consequently, extensive research has been devoted to MXenes in the quest for high-performance cathode materials that can effectively catalyze Li2O2 deposition and degradation. Among these efforts, controlling the surface terminal functional groups of MXenes has emerged as an effective strategy to enhance their catalytic efficiency. Theoretical calculations have demonstrated a catalytic activity trend for Ti3C2 as follows: Ti3C2O2 > Ti3C2F2 > Ti3C2(OH)2 > Ti3C2, highlighting the potential advantages of O-terminated Ti3C2 MXene for catalyzing both ORR and OER in LOBs[263]. Concurrently, experimental evidence has validated that introducing oxygen functional groups to the MXene surface effectively boosts its intrinsic activity[264-266]. Furthermore, incorporating heteroatoms has been identified as a promising approach to enhancing the catalytic performance of MXene. Another study involved the generation of a single-atom-doped Ti3C2 MXene catalyst with isolated semi-metallic selenium atoms[267]. These isolated selenium atom catalytic centers exhibit active behavior, substantially augmenting the intrinsic LiO2 absorption capacity. It fundamentally modifies the formation/decomposition mechanism of L2O2, thereby significantly improving redox kinetics and mitigating overpotentials. Constructing complexes has also been recognized as an effective technique to accelerate catalytic reaction kinetics[203,268,269]. Zheng et al. designed nitrogen-doped TiO2 on Ti3C2Tx nanosheets (N-TiO2/Ti3C2Tx) as the oxygen electrode catalyst for LOBs [Figure 12C][203]. The heterojunction between TiO2 and Ti3C2Tx nanosheets retained metallic properties and effectively facilitated Li+ and electron transfer within the electrode. More importantly, precise regulation of active sites at the N-TiO2/Ti3C2Tx heterojunction optimized the adsorption of LiO2 and Li2O2, thus accelerating the sluggish kinetics for both ORR and OER. As expected, N-TiO2/Ti3C2Tx catalyzed LOBs presented a superior specific capacity of 15,298 mAh g-1 and could run stably for over 200 cycles.
Other 2D catalysts
In addition to the catalysts described earlier, several other types of 2D materials have also been used in LOBs, such as MOFs, COFs, and g-C3N4. Of these, 2D MOF materials have been extensively investigated due to their notable characteristics, including high porosity, controllable large surface area, tunable structure and chemistry, and versatile chemical functionality achievable by substituting organic linkers and/or TMs. Yuan et al. were the pioneers in the application of 2D MOFs in aprotic LOBs[204]. They employed a straightforward ultrasonic method to prepare a range of MOFs as cathode catalysts, resulting in the desired ultrathin structure. Notably, 2D Mn-MOFs possessed the highest performance among all prepared 2D MOFs, exhibiting a higher initial discharge specific capacity of 9,464 mAh g-1 and exceptional cyclability over 200 cycles. This remarkable performance can be attributed to the highly electrocatalytic activity of Mn-O clusters in 2D Mn-MOFs for the decomposition of Li2O2 and LiOH, which enhances the reversibility and efficiency of LOBs. Lv et al. enhanced the intrinsic activity of 2D MOFs via both doping and spin state manipulation[270,271]. In the former approach, Ru atoms were incorporated into nickel-hexaiminotriphenylene (Ni-HTP), featuring quadrilateral Ni-N4 units[270]. The atomically dispersed Ru-N4 sites exhibited strong adsorption affinity for LiO2 intermediate due to their adjustable d-band center. This favored the generation of film-like Li2O2, promoting electron transfer and ion diffusion between the cathode and electrolyte and further facilitating Li2O2 decomposition during charging. This design allowed the LOBs with NiRu-HTP to achieve significantly reduced overpotential and remarkable cyclability. In the latter approach, the spin state of partial Ni2+ metal centers (t2g6eg2) within a conductive nickel catecholate framework (NiII-NCF) was manipulated to high-valence Ni3+ (t2g6eg1) to create NiIII-NCF[271]. This change of spin state enhanced Ni-O covalency in NiIII-NCF, promoting electron exchange between Ni sites and oxygen adsorbates and accelerating oxygen redox kinetics. The battery employing NiIII-NCF exhibited notably reduced discharge/charge voltage gaps, superior rate capability, and extended cycling stability. Furthermore, MXenes are frequently employed to form complexes with MOFs, enabling the catalysis of
Figure 13. (A) (a) Working principle of CMT@MXene bifunctional electrocatalyst with an electron hopping mechanism; (b) SEM image of CMT@MXene; (c) Charge-discharge profiles of samples[206]. Copyright 2023, Wiley-VCH. (B) (a) Molecular structures of Ni-TAPP-Co; (b) Top view of Ni-TAPP-Co; (c) SEM image of Ni-TAPP-Co; (d) Galvanostatic profiles for samples[207]. Copyright 2023, the American Association for the Advancement of Science.
As a semiconductor, the progress of g-C3N4 is constrained by its limited electronic conductivity and inadequate intrinsic activity for catalytic processes. In response to these challenges, strategies have been implemented to address these issues. To fix the problems mentioned, a conductive substrate, such as graphene, was first introduced to increase the number of electrons accumulated on the g-C3N4 surface, thereby enhancing the density of active sites[272,273]. However, the problem of low activity to g-C3N4 remained. To this end, efforts have been directed towards enhancing the catalytic activity of g-C3N4, encompassing approaches such as doping engineering, the integration of SAs, and the fabrication of composite materials in conjunction with other active substances. Upon investigating the ORR mechanism on the surfaces of g-C3N4 and its derivatives doped with S, O, and P, it was observed that S-doped g-C3N4 exhibited the highest intrinsic catalytic activity. DFT calculations revealed an overpotential of 0.41 V for S-doped g-C3N4 during the discharge process and 0.84 V for S-doped g-C3N4/Li, indicating the promising potential of S-doped
CONCLUSION AND OUTLOOK
MABs hold the promise of meeting the high energy density requirements of power batteries while offering benefits such as flexibility, portability, and enhanced safety. However, substantial challenges must be overcome if large-scale commercialization is to be achieved, including limited rate capabilities, high overpotential, insufficient cycling stability, uncontrolled side reactions, and unpredictable reaction mechanisms. This review begins by elucidating the operational mechanisms of ZABs and LOBs, which represent aqueous and nonaqueous batteries, respectively. The focus then shifts to 2D materials with significant potential as oxygen electrode catalysts in MABs. Material modification approaches and their respective functions are then summarized, and a comprehensive account of the latest advancements in 2D catalysts within MABs is provided. Confronting these challenges, the outlooks on the development of advanced MABs are as follows:
(I) Highly efficient bifunctional catalysts
The primary challenge lies in the rational design of effective, stable, and cost-efficient bifunctional catalysts that can replace unstable and expensive precious metal materials in MABs. Unlike traditional catalysts that mainly accelerate a single reaction, the cyclic nature of OER and ORR on the air electrode during charge and discharge necessitates catalysts for MABs to concurrently lower the energy barriers of both reactions. Therefore, the effective integration of active sites becomes a promising strategy. Unfortunately, despite substantial efforts, an optimal solution remains elusive. In pursuit of commercial viability, the second challenge pertains to catalyst stability, encompassing the catalyst's natural storage stability and its stability while functioning as a catalyst. In large-scale production, the ease of material storage becomes a pivotal consideration. For instance, MXenes, exceptional 2D carriers, are promising as efficient catalysts for both ZABs and LOBs when modified. Nevertheless, their susceptibility to oxidation in air or water solutions poses a significant hindrance, limiting their widespread application. Catalyst stability during reactions is another crucial concern. The causes of instability can result from the degradation of active sites and detachment from the electrode. The former is related to the reaction mechanism, which may be due to changes in the catalyst itself or result from the adsorption of by-products that cover the active site. The latter can be attributed to weak adhesion between catalyst and support and can be improved using binders or by allowing the catalyst to grow on the carrier. In terms of practical application, economic viability is unavoidable, which is also one of the reasons why precious metal catalysts are not suitable for widespread use. Similarly, intricate preparation processes, stringent conditions, and low yields can render catalysts impractical. The path ahead for catalyst development, therefore, remains long and complex.
(II) Means to regulate 2D nanomaterials
As for the modification means of 2D materials, they are mainly divided into two kinds according to their functions. One is the design of a hierarchical structure. The designed hierarchical structure is often rich in holes of different sizes, enhancing the contact between the catalyst and the electrolyte and facilitating the acceleration of the mass transfer and electron transfer as well. Moreover, such a stable structure can prevent the agglomeration of 2D structured materials during the reaction process to a certain extent. The second is surface environment adjustment of 2D nanomaterials, including the introduction of heteroatoms, the manipulation of defect structures, the adjustment of the interface, and so on. These methods have been demonstrated to change the Fermi level of 2D materials, and the newly constructed active site can affect the adsorption energy of the material surface with the reaction intermediates, thereby reducing the reaction energy barrier. However, the current research only focuses on one side, so the prepared catalysts still have various deficiencies. We propose to combine these two methods to improve the catalyst simultaneously in terms of multistage structure and atomic structure optimization. This will be the main design direction of 2D bifunctional catalysts in the future.
(III) Definite mechanism
The current understanding of the mechanism for MABs, although improving, remains partial and incomplete. Continued endeavors are essential to monitor the intricate processes of oxygen catalysis, aiming to attain a profound comprehension of the reaction mechanisms pertinent to both MABs and LOBs. The mechanism underpinning oxygen-catalyzed reactions is dynamic and subject to alteration by various factors such as temperature, electrolyte composition, and even electrode material, all of which complicate this area of research. Fortunately, with the development of new scientific equipment, more in-depth research can be performed. For instance, advanced in-situ characterization techniques, such as in-situ XRD, XPS, Raman, FTIR, and HRTEM, offer a real-time understanding of the evolution of crucial reaction intermediates. These techniques aid in unraveling essential information concerning reaction pathways, surface/interface behaviors, and performance degradation. We call on the premise of excellent battery system performance through the observation of intermediate products to deduce the experimental mechanism. Subsequently, a comprehensive analysis of a wealth of data is undertaken to thoroughly grasp the factors influencing the mechanism. This information is then looped back into the experimental design, encompassing catalyst development, to further enhance our understanding and guide future research directions.
(IV) Standard measurement
The battery performance is affected by various factors, including reaction temperatures, electrolytes, the area of electrodes, the mass loading of catalysts, or the concertation of O2. Hence, the establishment of criteria for assessing battery performance becomes imperative to identify the main factors. Only in this way can the comparison of catalysts be meaningful. Lastly, while conducting tests at low current levels is advantageous for comprehending reaction mechanisms, it is equally crucial to explore testing under high current conditions to cater to commercialization requirements.
In conclusion, the journey to develop advanced MABs is still far-reaching. We believe that this review provides some insights into high-efficiency and stable catalysts that can promote the rapid advancement of MABs.
DECLARATIONS
Authors’ contributions
Proposed the topic of this review: Kim JK, Park HS
Prepared the manuscript: Pei C, Zhang D, Kim J
Collectively discussed and revised the manuscript: Pei C, Zhang D, Yu X, Sim U, Park HS, Kim JK
Availability of data and materials
Not applicable.
Financial support and sponsorship
This research was supported by the "Regional Innovation Strategy (RIS)" through the National Research Foundation of Korea (NRF) funded by the Ministry of Education (MOE)(2021RIS-002) and the Korea Institute of Energy Technology Evaluation and Planning (KETEP) grant funded by the Korea government (MOTIE) (20214000000500, training program of CCUS for the green growth).
Conflicts of interest
All authors declared that there are no conflicts of interest.
Ethical approval and consent to participate
Not applicable.
Consent for publication
Not applicable.
Copyright
© The Author(s) 2024.
REFERENCES
1. Li Y, Lu J. Metal-air batteries: will they be the future electrochemical energy storage device of choice? ACS Energy Lett 2017;2:1370-7.
2. Chen Y, Xu J, He P, et al. Metal-air batteries: progress and perspective. Sci Bull 2022;67:2449-86.
3. Chang Z, Xu J, Zhang X. Recent progress in electrocatalyst for Li-O2 batteries. Adv Energy Mater 2017;7:1700875.
4. Cheng F, Chen J. Metal-air batteries: from oxygen reduction electrochemistry to cathode catalysts. Chem Soc Rev 2012;41:2172-92.
5. Zheng X, Yuan M, Zhao Y, et al. Status and prospects of MXene-based lithium-oxygen batteries: theoretical prediction and experimental modulation. Adv Energy Mater 2023;13:2204019.
6. Zhu X, Hu C, Amal R, Dai L, Lu X. Heteroatom-doped carbon catalysts for zinc-air batteries: progress, mechanism, and opportunities. Energy Environ Sci 2020;13:4536-63.
7. Jana M, Xu R, Cheng X, et al. Rational design of two-dimensional nanomaterials for lithium-sulfur batteries. Energy Environ Sci 2020;13:1049-75.
8. Jiang Y, Deng YP, Liang R, et al. Linker-compensated metal-organic framework with electron delocalized metal sites for bifunctional oxygen electrocatalysis. J Am Chem Soc 2022;144:4783-91.
9. Ji B, Gou J, Zheng Y, et al. Coordination chemistry of large-sized yttrium single-atom catalysts for oxygen reduction reaction. Adv Mater 2023;35:e2300381.
10. Sun Y, Liu X, Jiang Y, et al. Recent advances and challenges in divalent and multivalent metal electrodes for metal-air batteries. J Mater Chem A 2019;7:18183-208.
11. Abraham KM, Jiang Z. A polymer electrolyte-based rechargeable lithium/oxygen battery. J Electrochem Soc 1996;143:1-5.
12. Lai J, Xing Y, Chen N, Li L, Wu F, Chen R. Electrolytes for rechargeable lithium-air batteries. Angew Chem Int Ed 2020;59:2974-97.
13. Li X, Han G, Qian Z, et al. π-conjugation induced anchoring of ferrocene on graphdiyne enable shuttle-free redox mediation in lithium-oxygen batteries. Adv Sci 2022;9:2103964.
14. Cheng Y, Dou Y, Kan D, Wang Y, Wei Y. Electrocatalysis in Li-O2 battery over single-atom catalyst based on g-C3N4 substrate. Appl Surf Sci 2023;610:155481.
15. Pei C, Choi MS, Yu X, Xue H, Xia BY, Park HS. Recent progress in emerging metal and covalent organic frameworks for electrochemical and functional capacitors. J Mater Chem A 2021;9:8832-69.
16. Su D, Dou S, Wang G. Single crystalline Co3O4 nanocrystals exposed with different crystal planes for Li-O2 batteries. Sci Rep 2014;4:5767.
17. Thomas JM, Raja R, Lewis DW. Single-site heterogeneous catalysts. Angew Chem Int Ed 2005;44:6456-82.
18. Qiao B, Wang A, Yang X, et al. Single-atom catalysis of CO oxidation using Pt1/FeOx. Nat Chem 2011;3:634-41.
19. Novoselov KS, Geim AK, Morozov SV, et al. Electric field effect in atomically thin carbon films. Science 2004;306:666-9.
20. Jin H, Guo C, Liu X, et al. Emerging two-dimensional nanomaterials for electrocatalysis. Chem Rev 2018;118:6337-408.
21. Tang C, Wang HF, Chen X, et al. Topological defects in metal-free nanocarbon for oxygen electrocatalysis. Adv Mater 2016;28:6845-51.
22. Yu J, Dai Y, Zhang Z, et al. New nitrogen-doped graphitic carbon nanosheets with rich structural defects and hierarchical nanopores as efficient metal-free electrocatalysts for oxygen reduction reaction in Zn-air batteries. Chem Eng Sci 2022;259:117816.
23. Zhang L, Gu T, Lu K, Zhou L, Li D, Wang R. Engineering synergistic edge-N dipole in metal-free carbon nanoflakes toward intensified oxygen reduction electrocatalysis. Adv Funct Mater 2021;31:2103187.
24. Ma L, Liu W, Hu X, Lam PK, Zeng JR, Yu H. Ionothermal carbonization of biomass to construct sp2/sp3 carbon interface in N-doped biochar as efficient oxygen reduction electrocatalysts. Chem Eng J 2020;400:125969.
25. Lu T, Hu X, He J, et al. Aqueous/solid state Zn-air batteries based on N doped graphdiyne as efficient metal-free bifunctional catalyst. Nano Energy 2021;85:106024.
26. Lei W, Deng Y, Li G, et al. Two-dimensional phosphorus-doped carbon nanosheets with tunable porosity for oxygen reactions in zinc-air batteries. ACS Catal 2018;8:2464-72.
27. Li P, Jang H, Yuan B, Wu Z, Liu X, Cho J. Using lithium chloride as a medium to prepare N,P-codoped carbon nanosheets for oxygen reduction and evolution reactions. Inorg Chem Front 2019;6:417-22.
28. Wang Y, Xu N, He R, Peng L, Cai D, Qiao J. Large-scale defect-engineering tailored tri-doped graphene as a metal-free bifunctional catalyst for superior electrocatalytic oxygen reaction in rechargeable Zn-air battery. Appl Catal B 2021;285:119811.
29. Zheng X, Wu J, Cao X, et al. N-, P-, and S-doped graphene-like carbon catalysts derived from onium salts with enhanced oxygen chemisorption for Zn-air battery cathodes. Appl Catal B 2019;241:442-51.
30. Zheng D, Ci S, Cai P, Wang G, Wen Z. Nitrogen-doped carbon nanosheets encapsulating cobalt nanoparticle hybrids as high-performance bifunctional electrocatalysts. ChemElectroChem 2019;6:2683-8.
31. Liu P, Hu Y, Liu X, et al. Cu and Co nanoparticle-Co-decorated N-doped graphene nanosheets: a high efficiency bifunctional electrocatalyst for rechargeable Zn-air batteries. J Mater Chem A 2019;7:12851-8.
32. Yang ZK, Lin L, Xu AW. 2D nanoporous Fe-N/C nanosheets as highly efficient non-platinum electrocatalysts for oxygen reduction reaction in Zn-air battery. Small 2016;12:5710-9.
33. Shao C, Zhuang S, Zhang H, et al. Enhancement of mass transport for oxygen reduction reaction using petal-like porous Fe-NC nanosheet. Small 2021;17:e2006178.
34. Cui T, Wang YP, Ye T, et al. Engineering dual single-atom sites on 2D ultrathin N-doped carbon nanosheets attaining ultra-low-temperature zinc-air battery. Angew Chem Int Ed 2022;61:e202115219.
35. Zhang T, Wang F, Yang C, et al. Boosting ORR performance by single atomic divacancy Zn-N3C-C8 sites on ultrathin N-doped carbon nanosheets. Chem Catal 2022;2:836-52.
36. Chen Z, Peng X, Chen Z, et al. Mass production of sulfur-tuned single-atom catalysts for Zn-Air batteries. Adv Mater 2023;35:2209948.
37. Li Y, Ding Y, Zhang B, et al. N,O symmetric double coordination of an unsaturated Fe single-atom confined within a graphene framework for extraordinarily boosting oxygen reduction in Zn-air batteries. Energy Environ Sci 2023;16:2629-36.
38. Shang N, Wang C, Zhang X, et al. Atomically dispersed iron on nitrogen-decorated carbon for high-performance oxygen reduction and zinc-air batteries. Chem Eng J 2021;426:127345.
39. Li P, Wang H, Tan X, et al. Bifunctional electrocatalyst with CoN3 active sties dispersed on N-doped graphitic carbon nanosheets for ultrastable Zn-air batteries. Appl Catal B 2022;316:121674.
40. Zhou Y, Tao X, Chen G, et al. Multilayer stabilization for fabricating high-loading single-atom catalysts. Nat Commun 2020;11:5892.
41. Jin Q, Wang C, Guo Y, et al. Axial oxygen ligands regulating electronic and geometric structure of Zn-N-C sites to boost oxygen reduction reaction. Adv Sci 2023;10:e2302152.
42. Zhou S, Huang S, Wang X, et al. Activating the PdN4 single-atom sites for 4 electron oxygen reduction reaction via axial oxygen ligand modification. Chem Eng J 2023;472:145129.
43. Li YJ, Cui L, Da PF, et al. Multiscale structural engineering of Ni-doped CoO nanosheets for zinc-air batteries with high power density. Adv Mater 2018;30:e1804653.
44. Li Y, Talib SH, Liu D, et al. Improved oxygen evolution reaction performance in Co0.4Mn0.6O2 nanosheets through triple-doping (Cu, P, N) strategy and its application to Zn-air battery. Appl Catal B 2023;320:122023.
45. Tian Y, Liu X, Xu L, et al. Engineering crystallinity and oxygen vacancies of Co(II) oxide nanosheets for high performance and robust rechargeable Zn-air batteries. Adv Funct Mater 2021;31:2101239.
46. Liu P, Ran J, Xia B, Xi S, Gao D, Wang J. Bifunctional oxygen electrocatalyst of mesoporous Ni/NiO nanosheets for flexible rechargeable Zn-air batteries. Nanomicro Lett 2020;12:68.
47. Li Y, Cheng G, Zhou Z, et al. Shape-controlled synthesis of NiCo2O4-rGO as bifunctional electrocatalyst for Zn-air battery. ChemElectroChem 2019;6:4429-36.
48. Liu W, Bao J, Xu L, et al. NiCo2O4 ultrathin nanosheets with oxygen vacancies as bifunctional electrocatalysts for Zn-air battery. Appl Surf Sci 2019;478:552-9.
49. Yin J, Jin J, Liu H, et al. NiCo2O4-based nanosheets with uniform 4 nm mesopores for excellent Zn-air battery performance. Adv Mater 2020;32:e2001651.
50. Mondal S, Majee R, Arif Islam Q, Bhattacharyya S. 2D heterojunction between double perovskite oxide nanosheet and layered double hydroxide to promote rechargeable zinc-air battery performance. ChemElectroChem 2020;7:5005-12.
51. Han X, Li N, Baik JS, et al. Sulfur mismatch substitution in layered double hydroxides as efficient oxygen electrocatalysts for flexible zinc-air batteries. Adv Funct Mater 2023;33:2212233.
52. Cheng Y, Liao F, Shen W, et al. Carbon cloth supported cobalt phosphide as multifunctional catalysts for efficient overall water splitting and zinc-air batteries. Nanoscale 2017;9:18977-82.
53. Lan M, Xie C, Li B, et al. Two-dimensional cobalt sulfide/iron-nitrogen-carbon holey sheets with improved durability for oxygen electrocatalysis. ACS Appl Mater Interfaces 2022;14:11538-46.
54. Guo H, Gao X, Yu N, et al. Metallic state two-dimensional holey-structured Co3FeN nanosheets as stable and bifunctional electrocatalysts for zinc-air batteries. J Mater Chem A 2019;7:26549-56.
55. Han X, Li N, Xiong P, et al. Rhenium induced electronic structure modulation of Ni3S2/N-doped graphene for efficient trifunctional electrocatalysis. Compos Part B Eng 2022;234:109670.
56. Hao Y, Huang A, Han S, et al. Plasma-treated ultrathin ternary FePSe3 nanosheets as a bifunctional electrocatalyst for efficient zinc-air batteries. ACS Appl Mater Interfaces 2020;12:29393-403.
57. Yi M, Li N, Lu B, Li L, Zhu Z, Zhang J. Single-atom Pt decorated in heteroatom (N, B, and F)-doped ReS2 grown on Mo2CTx for efficient pH-universal hydrogen evolution reaction and flexible Zn-air batteries. Energy Stor Mater 2021;42:418-29.
58. Jiang Y, Deng YP, Liang R, et al. d-Orbital steered active sites through ligand editing on heterometal imidazole frameworks for rechargeable zinc-air battery. Nat Commun 2020;11:5858.
59. Cao Q, Wan L, Xu Z, et al. A fluorinated covalent organic framework with accelerated oxygen transfer nanochannels for high-performance zinc-air batteries. Adv Mater 2023;35:e2210550.
60. Niu WJ, He JZ, Wang YP, et al. A hybrid transition metal nanocrystal-embedded graphitic carbon nitride nanosheet system as a superior oxygen electrocatalyst for rechargeable Zn-air batteries. Nanoscale 2020;12:19644-54.
61. Zhang S, Shang N, Gao S, et al. Ultra dispersed Co supported on nitrogen-doped carbon: an efficient electrocatalyst for oxygen reduction reaction and Zn-air battery. Chem Eng Sci 2021;234:116442.
62. Wang X, Raghupathy RKM, Querebillo CJ, et al. Interfacial covalent bonds regulated electron-deficient 2D black phosphorus for electrocatalytic oxygen reactions. Adv Mater 2021;33:e2008752.
63. Chen L, Xu X, Yang W, Jia J. Recent advances in carbon-based electrocatalysts for oxygen reduction reaction. Chin Chem Lett 2020;31:626-34.
64. Katsounaros I, Cherevko S, Zeradjanin AR, Mayrhofer KJ. Oxygen electrochemistry as a cornerstone for sustainable energy conversion. Angew Chem Int Ed 2014;53:102-21.
65. Li FM, Huang L, Zaman S, et al. Corrosion chemistry of electrocatalysts. Adv Mater 2022;34:e2200840.
66. Xiong P, Tan J, Lee H, et al. Two-dimensional carbon-based heterostructures as bifunctional electrocatalysts for water splitting and metal-air batteries. Nano Mater Sci 2022; doi: 10.1016/j.nanoms.2022.10.001.
67. Sato Y, Yamada N, Kitano S, Kowalski D, Aoki Y, Habazaki H. High-corrosion-resistance mechanism of graphitized platelet-type carbon nanofibers in the OER in a concentrated alkaline electrolyte. J Mater Chem A 2022;10:8208-17.
68. Tang W, Mai J, Liu L, et al. Recent advances of bifunctional catalysts for zinc air batteries with stability considerations: from selecting materials to reconstruction. Nanoscale Adv 2023;5:4368-401.
69. Zhu J, Mu S. Defect engineering in carbon-based electrocatalysts: insight into intrinsic carbon defects. Adv Funct Mater 2020;30:2001097.
70. Yan D, Li Y, Huo J, Chen R, Dai L, Wang S. Defect chemistry of nonprecious-metal electrocatalysts for oxygen reactions. Adv Mater 2017;29:1606459.
71. Jiang Y, Yang L, Sun T, et al. Significant contribution of intrinsic carbon defects to oxygen reduction activity. ACS Catal 2015;5:6707-12.
72. Zeng Z, Yi L, He J, et al. Hierarchically porous carbon with pentagon defects as highly efficient catalyst for oxygen reduction and oxygen evolution reactions. J Mater Sci 2020;55:4780-91.
73. Chen D, Zhu J, Mu X, et al. Nitrogen-Doped carbon coupled FeNi3 intermetallic compound as advanced bifunctional electrocatalyst for OER, ORR and zn-air batteries. Appl Catal B 2020;268:118729.
74. Zhang LH, Shi Y, Wang Y, Shiju NR. Nanocarbon catalysts: recent understanding regarding the active sites. Adv Sci 2020;7:1902126.
75. Tao L, Wang Q, Dou S, et al. Edge-rich and dopant-free graphene as a highly efficient metal-free electrocatalyst for the oxygen reduction reaction. Chem Commun 2016;52:2764-7.
76. Yang H, Han X, Douka AI, et al. Advanced oxygen electrocatalysis in energy conversion and storage. Adv Funct Mater 2021;31:2007602.
77. Gong K, Du F, Xia Z, Durstock M, Dai L. Nitrogen-doped carbon nanotube arrays with high electrocatalytic activity for oxygen reduction. Science 2009;323:760-4.
78. Zhang J, Zhao Z, Xia Z, Dai L. A metal-free bifunctional electrocatalyst for oxygen reduction and oxygen evolution reactions. Nat Nanotechnol 2015;10:444-52.
79. Wohlgemuth S, White RJ, Willinger M, Titirici M, Antonietti M. A one-pot hydrothermal synthesis of sulfur and nitrogen doped carbon aerogels with enhanced electrocatalytic activity in the oxygen reduction reaction. Green Chem 2012;14:1515-23.
80. Jiao Y, Zheng Y, Jaroniec M, Qiao SZ. Origin of the electrocatalytic oxygen reduction activity of graphene-based catalysts: a roadmap to achieve the best performance. J Am Chem Soc 2014;136:4394-403.
81. Zhang S, Cai Y, He H, et al. Heteroatom doped graphdiyne as efficient metal-free electrocatalyst for oxygen reduction reaction in alkaline medium. J Mater Chem A 2016;4:4738-44.
82. Feng Z, Ma Y, Li Y, Li R, Tang Y, Dai X. Charge-compensated co-doping of graphdiyne with boron and nitrogen to form metal-free electrocatalysts for the oxygen reduction reaction. Phys Chem Chem Phys 2020;22:1493-501.
83. Li R, Wei Z, Gou X. Nitrogen and phosphorus dual-doped graphene/carbon nanosheets as bifunctional electrocatalysts for oxygen reduction and evolution. ACS Catal 2015;5:4133-42.
84. Hu C, Liang Q, Yang Y, et al. Conductivity-enhanced porous N/P co-doped metal-free carbon significantly enhances oxygen reduction kinetics for aqueous/flexible zinc-air batteries. J Colloid Interface Sci 2023;633:500-10.
85. Li M, Wang K, Lv Q. N,P-co-Doped Graphdiyne as efficient metal-free catalysts for oxygen reduction reaction. Chem Res Chin Univ 2021;37:1283-8.
86. Choi CH, Park SH, Woo SI. Binary and ternary doping of nitrogen, boron, and phosphorus into carbon for enhancing electrochemical oxygen reduction activity. ACS Nano 2012;6:7084-91.
87. Huang B, Liu Y, Huang X, Xie Z. Multiple heteroatom-doped few-layer carbons for the electrochemical oxygen reduction reaction. J Mater Chem A 2018;6:22277-86.
88. Wang J, Hu H, Zhang H, et al. Regulating the catalytically active sites in low-cost and earth-abundant 3D transition-metal-based electrode materials for high-performance zinc-air batteries. Energy Fuels 2021;35:6483-503.
89. Wei L, Ang EH, Yang Y, et al. Recent advances of transition metal based bifunctional electrocatalysts for rechargeable zinc-air batteries. J Power Sources 2020;477:228696.
90. Yang D, Chen D, Jiang Y, et al. Carbon-based materials for all-solid-state zinc-air batteries. Carbon Energy 2021;3:50-65.
91. Fu G, Yan X, Chen Y, et al. Boosting bifunctional oxygen electrocatalysis with 3D graphene aerogel-supported Ni/MnO particles. Adv Mater 2018;30:1704609.
92. Fu G, Wang J, Chen Y, et al. Exploring indium-based ternary thiospinel as conceivable high-potential air-cathode for rechargeable Zn-air batteries. Adv Energy Mater 2018;8:1802263.
93. Liu Y, Chen Z, Li Z, et al. CoNi nanoalloy-Co-N4 composite active sites embedded in hierarchical porous carbon as bi-functional catalysts for flexible Zn-air battery. Nano Energy 2022;99:107325.
94. Du L, Luo L, Feng Z, et al. Nitrogen-doped graphitized carbon shell encapsulated NiFe nanoparticles: a highly durable oxygen evolution catalyst. Nano Energy 2017;39:245-52.
95. Fu Y, Yu H, Jiang C, et al. NiCo alloy nanoparticles decorated on N-doped carbon nanofibers as highly active and durable oxygen electrocatalyst. Adv Funct Mater 2018;28:1705094.
96. Xu X, Xie J, Liu B, et al. PBA-derived FeCo alloy with core-shell structure embedded in 2D N-doped ultrathin carbon sheets as a bifunctional catalyst for rechargeable Zn-air batteries. Appl Catal B 2022;316:121687.
97. Yang XF, Wang A, Qiao B, Li J, Liu J, Zhang T. Single-atom catalysts: a new frontier in heterogeneous catalysis. Acc Chem Res 2013;46:1740-8.
98. Chen Y, Ji S, Chen C, Peng Q, Wang D, Li Y. Single-atom catalysts: synthetic strategies and electrochemical applications. Joule 2018;2:1242-64.
99. Han J, Bao H, Wang J, et al. 3D N-doped ordered mesoporous carbon supported single-atom Fe-N-C catalysts with superior performance for oxygen reduction reaction and zinc-air battery. Appl Catal B 2021;280:119411.
100. Nørskov JK, Rossmeisl J, Logadottir A, et al. Origin of the overpotential for oxygen reduction at a fuel-cell cathode. J Phys Chem B 2004;108:17886-92.
101. Peng H, Liu F, Liu X, et al. Effect of transition metals on the structure and performance of the doped carbon catalysts derived from polyaniline and melamine for ORR application. ACS Catal 2014;4:3797-805.
102. Zheng Y, Yang D, Kweun JM, et al. Rational design of common transition metal-nitrogen-carbon catalysts for oxygen reduction reaction in fuel cells. Nano Energy 2016;30:443-9.
103. Zhao C, Li B, Liu J, Zhang Q. Intrinsische elektrokatalytische aktivitätssteuerung von M-N-C-einzelatom-katalysatoren für die sauerstoffreduktionsreaktion. Angew Chemie Int Ed 2021;133:4496-512.
104. Shang H, Zhou X, Dong J, et al. Engineering unsymmetrically coordinated Cu-S1N3 single atom sites with enhanced oxygen reduction activity. Nat Commun 2020;11:3049.
105. Yu L, Li Y, Ruan Y. Dynamic control of sacrificial bond transformation in the Fe-N-C single-atom catalyst for molecular oxygen reduction. Angew Chem Int Ed 2021;60:25296-301.
106. Li L, Chen Y, Xing H, et al. Single-atom Fe-N5 catalyst for high-performance zinc-air batteries. Nano Res 2022;15:8056-64.
107. Hu L, Dai C, Chen L, et al. Metal-triazolate-framework-derived FeN4Cl1 single-atom catalysts with hierarchical porosity for the oxygen reduction reaction. Angew Chemie Int Ed 2021;133:27530-5.
108. Peng L, Yang J, Yang Y, et al. Mesopore-rich Fe-N-C catalyst with FeN4-O-NC single-atom sites delivers remarkable oxygen reduction reaction performance in alkaline media. Adv Mater 2022;34:e2202544.
109. Li X, Liu J, Cai Q, Kan Z, Liu S, Zhao J. Engineering d-band center of iron single atom site through boron incorporation to trigger the efficient bifunctional oxygen electrocatalysis. J Colloid Interface Sci 2022;628:331-42.
110. Wang X, An Y, Liu L, et al. Atomically dispersed pentacoordinated-zirconium catalyst with axial oxygen ligand for oxygen reduction reaction. Angew Chem Int Ed 2022;61:e202209746.
111. Seh ZW, Kibsgaard J, Dickens CF, Chorkendorff I, Nørskov JK, Jaramillo TF. Combining theory and experiment in electrocatalysis: insights into materials design. Science 2017;355:eaad4998.
112. Li H, Zhang Y, Hu X, Liu W, Chen J, Yu H. Metal-organic framework templated Pd@PdO-Co3O4 nanocubes as an efficient bifunctional oxygen electrocatalyst. Adv Energy Mater 2018;8:1702734.
113. Dai Y, Yu J, Wang J, et al. Bridging the charge accumulation and high reaction order for high-rate oxygen evolution and long stable Zn-air batteries. Adv Funct Mater 2022;32:2111989.
114. Lee DU, Xu P, Cano ZP, Kashkooli AG, Park MG, Chen Z. Recent progress and perspectives on bi-functional oxygen electrocatalysts for advanced rechargeable metal-air batteries. J Mater Chem A 2016;4:7107-34.
115. Lu Z, Wang H, Kong D, et al. Electrochemical tuning of layered lithium transition metal oxides for improvement of oxygen evolution reaction. Nat Commun 2014;5:4345.
116. He Y, Zhang J, He G, et al. Ultrathin Co3O4 nanofilm as an efficient bifunctional catalyst for oxygen evolution and reduction reaction in rechargeable zinc-air batteries. Nanoscale 2017;9:8623-30.
117. Yan K, Qin J, Lin J, et al. Probing the active sites of Co3O4 for the acidic oxygen evolution reaction by modulating the Co2+/Co3+ ratio. J Mater Chem A 2018;6:5678-86.
118. Zhang X, Liu R, Zang Y, et al. Co/CoO nanoparticles immobilized on Co-N-doped carbon as trifunctional electrocatalysts for oxygen reduction, oxygen evolution and hydrogen evolution reactions. Chem Commun 2016;52:5946-9.
119. Li Y, Zhong C, Liu J, et al. Atomically thin mesoporous Co3O4 layers strongly coupled with N-rGO nanosheets as high-performance bifunctional catalysts for 1D knittable zinc-air batteries. Adv Mater 2018;30:1703657.
120. Zheng Z, Huang L, Zhou Y, Hu X, Ni X. Large-scale synthesis of mesoporous CoO-doped NiO hexagonal nanoplatelets with improved electrochemical performance. Solid State Sci 2009;11:1439-43.
121. Tan P, Chen B, Xu H, Cai W, He W, Ni M. Growth of Al and Co co-doped NiO nanosheets on carbon cloth as the air electrode for Zn-air batteries with high cycling stability. Electrochim Acta 2018;290:21-9.
122. Qian J, Guo X, Wang T, Liu P, Zhang H, Gao D. Bifunctional porous Co-doped NiO nanoflowers electrocatalysts for rechargeable zinc-air batteries. Appl Catal B 2019;250:71-7.
123. Tian Y, Xu L, Qiu J, Liu X, Zhang S. Rational design of sustainable transition metal-based bifunctional electrocatalysts for oxygen reduction and evolution reactions. Sustain Mater Technol 2020;25:e00204.
124. Ao K, Shi J, Zhang X, Daoud WA. Tuning oxygen vacancies in spinel nanosheets for binder-free oxygen cathodes with superior catalytic activity in zinc-air batteries. J Power Sources 2022;521:230918.
125. Jin W, Chen J, Liu B, et al. Oxygen vacancy-rich in-doped CoO/CoP heterostructure as an effective air cathode for rechargeable Zn-air batteries. Small 2019;15:e1904210.
126. Zhu K, Shi F, Zhu X, Yang W. The roles of oxygen vacancies in electrocatalytic oxygen evolution reaction. Nano Energy 2020;73:104761.
127. Ji Q, Bi L, Zhang J, Cao H, Zhao XS. The role of oxygen vacancies of ABO3 perovskite oxides in the oxygen reduction reaction. Energy Environ Sci 2020;13:1408-28.
128. Wang N, Ning S, Yu X, et al. Graphene composites with Ru-RuO2 heterostructures: highly efficient Mott-Schottky-type electrocatalysts for pH-universal water splitting and flexible zinc-air batteries. Appl Catal B 2022;302:120838.
129. Dong Q, Ji S, Wang H, Linkov V, Wang R. Oxygen spillover effect at Cu/Fe2O3 heterointerfaces to enhance oxygen electrocatalytic reactions for rechargeable Zn-air batteries. ACS Appl Mater Interfaces 2022;14:51222-33.
130. Guo X, Liu S, Wan X, et al. Controllable solid-phase fabrication of an Fe2O3/Fe5C2/Fe-N-C electrocatalyst toward optimizing the oxygen reduction reaction in zinc-air batteries. Nano Lett 2022;22:4879-87.
131. Park J, Risch M, Nam G, et al. Single crystalline pyrochlore nanoparticles with metallic conduction as efficient bi-functional oxygen electrocatalysts for Zn-air batteries. Energy Environ Sci 2017;10:129-36.
132. Yan Y, Xia BY, Zhao B, Wang X. A review on noble-metal-free bifunctional heterogeneous catalysts for overall electrochemical water splitting. J Mater Chem A 2016;4:17587-603.
133. Li C, Han X, Cheng F, Hu Y, Chen C, Chen J. Phase and composition controllable synthesis of cobalt manganese spinel nanoparticles towards efficient oxygen electrocatalysis. Nat Commun 2015;6:7345.
134. Ni B, Ouyang C, Xu X, Zhuang J, Wang X. Modifying commercial carbon with trace amounts of ZIF to prepare derivatives with superior ORR activities. Adv Mater 2017;29:1701354.
135. Xu W, Lu Z, Sun X, Jiang L, Duan X. Superwetting electrodes for gas-involving electrocatalysis. Acc Chem Res 2018;51:1590-8.
136. Wang H, Zhou M, Choudhury P, Luo H. Perovskite oxides as bifunctional oxygen electrocatalysts for oxygen evolution/reduction reactions - a mini review. Appl Mater Today 2019;16:56-71.
137. Liu K, Li J, Wang Q, et al. Designed synthesis of LaCoO3/N-doped reduced graphene oxide nanohybrid as an efficient bifunctional electrocatalyst for ORR and OER in alkaline medium. J Alloys Compd 2017;725:260-9.
138. Ran J, Wang T, Zhang J, et al. Modulation of electronics of oxide perovskites by sulfur doping for electrocatalysis in rechargeable Zn-air batteries. Chem Mater 2020;32:3439-46.
139. Hui X, Zhang P, Li J, et al. In situ integrating highly ionic conductive LDH-array@PVA gel electrolyte and MXene/Zn anode for dendrite-free high-performance flexible Zn-air batteries. Adv Energy Mate 2022;12:2201393.
140. Zhou D, Xiong X, Cai Z, et al. Flame-engraved nickel-iron layered double hydroxide nanosheets for boosting oxygen evolution reactivity. Small Methods 2018;2:1800083.
141. Yan D, Wang W, Luo X, Chen C, Zeng Y, Zhu Z. NiCo2O4 with oxygen vacancies as better performance electrode material for supercapacitor. Chem Eng J 2018;334:864-72.
142. Ambriz-peláez O, Béjar J, Ramos-castillo C, Guerra-balcázar M, Álvarez-contreras L, Arjona N. Defected NiFe layered double hydroxides on N-doped carbon nanotubes as efficient bifunctional electrocatalyst for rechargeable zinc-air batteries. Appl Surface Sci 2022;601:154253.
143. Wang T, Nam G, Jin Y, et al. NiFe (Oxy) hydroxides derived from NiFe disulfides as an efficient oxygen evolution catalyst for rechargeable Zn-air batteries: the effect of surface S residues. Adv Mater 2018;30:e1800757.
144. Jia Z, Shang J, Xue K, et al. N-doped nanocarbon inserted NiCo-LDH nanoplates on NF with high OER/ORR performances for zinc-air battery. ChemCatChem 2023;15:e202201469.
145. Kumar DB, Nie W, Jiang Z, Lee J, Maiyalagan T. Recent progress in transition metal carbides and nitrides based composites as bifunctional oxygen electrocatalyst for zinc air batteries. J Alloys Compd 2023;960:170828.
146. Yu X, Zhou T, Ge J, Wu C. Recent advances on the modulation of electrocatalysts based on transition metal nitrides for the rechargeable Zn-air battery. ACS Mater Lett 2020;2:1423-34.
147. Liang Y, Gong Q, Sun X, Xu N, Gong P, Qiao J. Rational fabrication of thin-layered NiCo2S4 loaded graphene as bifunctional non-oxide catalyst for rechargeable zinc-air batteries. Electrochim Acta 2020;342:136108.
148. Xu R, Xu Z, Zhang X, Ling Y, Li M, Yang Z. Cobalt-doped tungsten sulfides as stable and efficient air electrodes for rechargeable zinc-air batteries. ChemElectroChem 2020;7:148-54.
149. Wu Z, Wang H, Xiong P, et al. Molecularly thin nitride sheets stabilized by titanium carbide as efficient bifunctional electrocatalysts for fiber-shaped rechargeable zinc-air batteries. Nano Lett 2020;20:2892-8.
150. Luo M, Koper MTM. A kinetic descriptor for the electrolyte effect on the oxygen reduction kinetics on Pt(111). Nat Catal 2022;5:615-23.
151. Yu J, Li Z, Liu T, et al. Morphology control and electronic tailoring of CoxAy (A = P, S, Se) electrocatalysts for water splitting. Chem Eng J 2023;460:141674.
152. Sun Y, Guan Y, Wu X, et al. ZIF-derived "senbei"-like Co9S8/CeO2/Co heterostructural nitrogen-doped carbon nanosheets as bifunctional oxygen electrocatalysts for Zn-air batteries. Nanoscale 2021;13:3227-36.
153. Naguib M, Kurtoglu M, Presser V, et al. Two-dimensional nanocrystals produced by exfoliation of Ti3AlC2. Adv Mater 2011;23:4248-53.
154. Lukatskaya MR, Mashtalir O, Ren CE, et al. Cation intercalation and high volumetric capacitance of two-dimensional titanium carbide. Science 2013;341:1502-5.
155. Ghidiu M, Lukatskaya MR, Zhao MQ, Gogotsi Y, Barsoum MW. Conductive two-dimensional titanium carbide 'clay' with high volumetric capacitance. Nature 2014;516:78-81.
156. Yue L, Chen L, Wang X, et al. Ni/Co-MOF@aminated MXene hierarchical electrodes for high-stability supercapacitors. Chem Eng J 2023;451:138687.
157. Seh ZW, Fredrickson KD, Anasori B, et al. Two-dimensional molybdenum carbide (MXene) as an efficient electrocatalyst for hydrogen evolution. ACS Energy Lett 2016;1:589-94.
158. kong W, Li L, Yu X, et al. Platinum nickel alloy-MXene catalyst with inverse opal structure for enhanced hydrogen evolution in both acidic and alkaline solutions. Nano Res 2023;16:195-201.
159. Chen Y, Yao H, Kong F, et al. V2C MXene synergistically coupling FeNi LDH nanosheets for boosting oxygen evolution reaction. Appl Catal B 2021;297:120474.
160. Zou H, He B, Kuang P, Yu J, Fan K. Metal-organic framework-derived nickel-cobalt sulfide on ultrathin mxene nanosheets for electrocatalytic oxygen evolution. ACS Appl Mater Interfaces 2018;10:22311-9.
161. Franco A, Salatti-dorado JÁ, García-caballero V, et al. A 2D copper-imidazolate framework without thermal treatment as an efficient ORR electrocatalyst for Zn-air batteries. J Mater Chem A 2022;10:24590-7.
162. Zhao L, Dong B, Li S, et al. Interdiffusion reaction-assisted hybridization of two-dimensional metal-organic frameworks and Ti3C2Tx nanosheets for electrocatalytic oxygen evolution. ACS Nano 2017;11:5800-7.
163. Zheng X, Cao Y, Liu D, et al. Bimetallic metal-organic-framework/reduced graphene oxide composites as bifunctional electrocatalysts for rechargeable Zn-air batteries. ACS Appl Mater Interfaces 2019;11:15662-9.
164. Liu M, Liu S, Cui CX, et al. Construction of catalytic covalent organic frameworks with redox-active sites for the oxygen reduction and the oxygen evolution reaction. Angew Chem Int Ed 2022;61:e202213522.
165. Yang S, Li X, Tan T, et al. A fully-conjugated covalent organic framework-derived carbon supporting ultra-close single atom sites for ORR. Appl Catal B 2022;307:121147.
166. Han X, Zhang W, Ma X, et al. Identifying the activation of bimetallic sites in NiCo2S4@g-C3N4-CNT hybrid electrocatalysts for synergistic oxygen reduction and evolution. Adv Mater 2019;31:e1808281.
167. zheng J, Kang T, Liu B, Wang P, Li H, Yang M. N-doped carbon nanotubes encapsulated with FeNi nanoparticles derived from defect-rich, molecule-doped 3D g-C3N4 as an efficient bifunctional electrocatalyst for rechargeable zinc-air batteries. J Mater Chem A 2022;10:9911-21.
168. Zhu X, Zhang J, Wang Y, et al. Achieving high-performance oxygen reduction catalyst and Zn-air battery through a synergistic nitrogen doping strategy. Energy Technol 2022;10:2200602.
169. Wang C, Zhao H, Wang J, et al. Atomic Fe hetero-layered coordination between g-C3N4 and graphene nanomeshes enhances the ORR electrocatalytic performance of zinc-air batteries. J Mater Chem A 2019;7:1451-8.
170. Jiang Q, Gan C, Wu X, Liu Z, Tang J. Facile synthesis of black phosphorus directly grown on carbon paper as an efficient OER electrocatalyst: role of interfacial charge transfer and induced local charge distribution. Adv owder Technol 2022;33:103371.
171. Kang J, Wells SA, Wood JD, et al. Stable aqueous dispersions of optically and electronically active phosphorene. Proc Natl Acad Sci USA 2016;113:11688-93.
172. Ling X, Wang H, Huang S, Xia F, Dresselhaus MS. The renaissance of black phosphorus. Proc Natl Acad Sci USA 2015;112:4523-30.
173. Yang S, Zhang K, Ricciardulli AG, et al. A delamination strategy for thinly layered defect-free high-mobility black phosphorus flakes. Angew Chem Int Ed 2018;57:4677-81.
174. Jung I, Kwon HJ, Kim M, et al. Rapid oxygen diffusive lithium-oxygen batteries using a restacking-inhibited, free-standing graphene cathode film. J Mater Chem A 2019;7:10397-404.
175. Huang H, Zhu J, Zhang W, et al. Controllable codoping of nitrogen and sulfur in graphene for highly efficient Li-Oxygen batteries and direct methanol fuel cells. Chem Mater 2016;28:1737-45.
176. Zhang J, Luo X, Li X, et al. Two-dimensional boron and nitrogen dual-doped graphitic carbon as an efficient metal-free cathodic electrocatalyst for lithium-air batteries. ChemElectroChem 2021;8:949-56.
177. Han J, Guo X, Ito Y, et al. Effect of chemical doping on cathodic performance of bicontinuous nanoporous graphene for Li-O2 batteries. Adv Energy Mater 2016;6:1501870.
178. Xiao F, Meng Y, Lin Z, et al. Highly boron-doped holey graphene for lithium oxygen batteries with enhanced electrochemical performance. Carbon 2022;189:404-12.
179. Dai W, Liu Y, Wang M, et al. Monodispersed ruthenium nanoparticles on nitrogen-doped reduced graphene oxide for an efficient lithium-oxygen battery. ACS Appl Mater Interfaces 2021;13:19915-26.
180. Wu F, Xing Y, Zeng X, et al. Platinum-coated hollow graphene nanocages as cathode used in lithium-oxygen batteries. Adv Funct Mater 2016;26:7626-33.
181. Lu J, Lee YJ, Luo X, et al. A lithium-oxygen battery based on lithium superoxide. Nature 2016;529:377-82.
182. Chen Y, Zhang Q, Zhang Z, et al. Two better than one: cobalt-copper bimetallic yolk-shell nanoparticles supported on graphene as excellent cathode catalysts for Li-O2 batteries. J Mater Chem A 2015;3:17874-9.
183. Xu SM, Liang X, Wu XY, et al. Multistaged discharge constructing heterostructure with enhanced solid-solution behavior for long-life lithium-oxygen batteries. Nat Commun 2019;10:5810.
184. Wang P, Ren Y, Wang R, et al. Atomically dispersed cobalt catalyst anchored on nitrogen-doped carbon nanosheets for lithium-oxygen batteries. Nat Commun 2020;11:1576.
185. Zhang W, Zheng J, Wang R, et al. Water-trapping single-atom Co-N4/graphene triggering direct 4e- LiOH chemistry for rechargeable aprotic Li-O2 batteries. Small 2023;19:e2301391.
186. Liu M, Li J, Chi B, et al. Integration of single Co atoms and Ru nanoclusters boosts the cathodic performance of nitrogen-doped 3D graphene in lithium-oxygen batteries. J Mater Chem A 2021;9:10747-57.
187. Zhang Y, Zhang S, Ma J, Chen X, Nan C, Chen C. Single-atom-mediated spinel octahedral structures for elevated performances of Li-oxygen batteries. Angew Chem Int Ed 2023;62:e202218926.
188. Sun Z, Cao X, Tian M, et al. Synergized multimetal oxides with amorphous/crystalline heterostructure as efficient electrocatalysts for lithium-oxygen batteries. Adv Energy Mater 2021;11:2100110.
189. Kim YS, Lee G, Sung M, Kim D. Orthorhombically distorted perovskite SeZnO3 nanosheets as an electrocatalyst for lithium-oxygen batteries. Chem Eng J 2021;406:126896.
190. Gao R, Chen Q, Zhang W, et al. Oxygen defects-engineered LaFeO3-x nanosheets as efficient electrocatalysts for lithium-oxygen battery. J Catal 2020;384:199-207.
191. Ju B, Song HJ, Lee G, Sung M, Kim D. Nickel disulfide nanosheet as promising cathode electrocatalyst for long-life lithium-oxygen batteries. Energy Stor Mater 2020;24:594-601.
192. Sennu P, Christy M, Aravindan V, Lee Y, Nahm KS, Lee Y. Two-dimensional mesoporous cobalt sulfide nanosheets as a superior anode for a Li-ion battery and a bifunctional electrocatalyst for the Li-O2 system. Chem Mater 2015;27:5726-35.
193. Wang G, Li Y, Shi L, Qian R, Wen Z. Realizing the growth of nano-network Li2O2 film on defect-rich holey Co9S8 nanosheets for Li-O2 battery. Chem Eng J 2020;396:125228.
194. Li D, Zhao L, Wang J, Yang C. Tailoring the d-Band Center over isomorphism pyrite catalyst for optimized intrinsic affinity to intermediates in lithium-oxygen batteries. Adv Energy Mater 2023;13:2204057.
195. Long J, Hou Z, Shu C, et al. Free-standing three-dimensional CuCo2S4 nanosheet array with high catalytic activity as an efficient oxygen electrode for lithium-oxygen batteries. ACS Appl Mater Interfaces 2019;11:3834-42.
196. Feng J, Wang H, Guo L, et al. Stacking surface derived catalytic capability and by-product prevention for high efficient two dimensional Bi2Te3 cathode catalyst in Li-oxygen batteries. Appl Catal B 2022;318:121844.
197. Zhang P, Lu X, Huang Y, et al. MoS2 nanosheets decorated with gold nanoparticles for rechargeable Li-O2 batteries. J Mater Chem A 2015;3:14562-6.
198. Li D, Zhao L, Xia Q, et al. Activating MoS2 nanoflakes via sulfur defect engineering wrapped on CNTs for stable and efficient Li-O2 batteries. Adv Funct Mater 2022;32:2108153.
199. Cao X, Zhang Y, Lu C, et al. Synergistic dual atomic sites with localized electronic modulation enable high-performance lithium-oxygen batteries. Chem Eng J 2023;466:143351.
200. Hu A, Shu C, Xu C, et al. Interface-engineered metallic 1T-MoS2 nanosheet array induced via palladium doping enabling catalysis enhancement for lithium-oxygen battery. Chem Eng J 2020;382:122854.
201. Zhang G, Liu C, Guo L, Liu R, Miao L, Dang F. Electronic “bridge” construction via Ag intercalation to diminish catalytic anisotropy for 2D tin diselenide cathode catalyst in lithium-oxygen batteries. Adv Energy Mater 2022;12:2200791.
202. Huang HB, Luo SH, Liu CL, Yi TF, Zhai YC. High-Surface-area and porous Co2P nanosheets as cost-effective cathode catalysts for Li-O2 batteries. ACS Appl Mater Interfaces 2018;10:21281-90.
203. Zheng X, Yuan M, Guo D, et al. Theoretical design and structural modulation of a surface-functionalized Ti3C2Tx MXene-based heterojunction electrocatalyst for a Li-oxygen battery. ACS Nano 2022;16:4487-99.
204. Yuan M, Wang R, Fu W, et al. Ultrathin two-dimensional metal-organic framework nanosheets with the inherent open active sites as electrocatalysts in aprotic Li-O2 batteries. ACS Appl Mater Interfaces 2019;11:11403-13.
205. Zhang W, Tang S, Chen Z, et al. The controllable construction of nanochannel in two-dimensional lamellar film for efficient oxygen reduction reaction and lithium-oxygen batteries. Chem Eng J 2022;430:132489.
206. Nam S, Mahato M, Matthews K, et al. Bimetal organic framework-Ti3C2Tx MXene with metalloporphyrin electrocatalyst for lithium-oxygen batteries. Adv Funct Mater 2023;33:2210702.
207. Ke SW, Li W, Gu Y, et al. Covalent organic frameworks with Ni-Bis(dithiolene) and Co-porphyrin units as bifunctional catalysts for Li-O2 batteries. Sci Adv 2023;9:eadf2398.
208. Zhao W, Wang J, Yin R, et al. Single-atom Pt supported on holey ultrathin g-C3N4 nanosheets as efficient catalyst for Li-O2 batteries. J Colloid Interface Sci 2020;564:28-36.
209. Guo Q, Zhang C, Zhang C, et al. Co3O4 modified Ag/g-C3N4 composite as a bifunctional cathode for lithium-oxygen battery. J Energy Chem 2020;41:185-93.
210. Li Y, Wang J, Li X, Geng D, Li R, Sun X. Superior energy capacity of graphene nanosheets for a nonaqueous lithium-oxygen battery. Chem Commun 2011;47:9438-40.
211. Wu G, Mack NH, Gao W, et al. Nitrogen-doped graphene-rich catalysts derived from heteroatom polymers for oxygen reduction in nonaqueous lithium-O2 battery cathodes. ACS Nano 2012;6:9764-76.
212. Liu M, Zhu X, Song Y, et al. Bifunctional edge-rich nitrogen doped porous carbon for activating oxygen and sulfur. Adv Funct Mater 2023;33:2213395.
213. Lin Y, Moitoso B, Martinez-Martinez C, et al. Ultrahigh-capacity lithium-oxygen batteries enabled by dry-pressed holey graphene air cathodes. Nano Lett 2017;17:3252-60.
214. Shui J, Lin Y, Connell JW, Xu J, Fan X, Dai L. Nitrogen-doped holey graphene for high-performance rechargeable Li-O2 batteries. ACS Energy Lett 2016;1:260-5.
215. Ren X, Zhu J, Du F, Liu J, Zhang W. B-doped graphene as catalyst to improve charge rate of lithium-air battery. J Phys Chem C 2014;118:22412-8.
216. Zoubi W, Putri RAK, Abukhadra MR, Ko YG. Recent experimental and theoretical advances in the design and science of high-entropy alloy nanoparticles. Nano Energy 2023;110:108362.
217. Kumar S, Kumar S, Kumar D, Sathish N, Singh A, Goswami M. Reduced graphene oxide and Pd nanocomposite as a catalyst for oxygen reduction reaction in rechargeable Li-oxygen battery. ChemistrySelect 2019;4:8404-9.
218. Su D, Han Seo D, Ju Y, et al. Ruthenium nanocrystal decorated vertical graphene nanosheets@Ni foam as highly efficient cathode catalysts for lithium-oxygen batteries. NPG Asia Mater 2016;8:e286.
219. Ye SJ, Kim DY, Kim DW, Park OO, Kang Y. Facile synthesis of palladium nanodendrites supported on graphene nanoplatelets: an efficient catalyst for low overpotentials in lithium-oxygen batteries. J Mater Chem A 2016;4:578-86.
220. Zhou W, Cheng Y, Yang X, et al. Iridium incorporated into deoxygenated hierarchical graphene as a high-performance cathode for rechargeable Li-O2 batteries. J Mater Chem A 2015;3:14556-61.
221. Bi X, Li M, Liu C, et al. Cation Additive enabled rechargeable LiOH-based lithium-oxygen batteries. Angew Chem Int Ed 2020;59:22978-82.
223. Zhang M, Zou L, Yang C, Chen Y, Shen Z, Bo C. An all-nanosheet OER/ORR bifunctional electrocatalyst for both aprotic and aqueous Li-O2 batteries. Nanoscale 2019;11:2855-62.
224. Tang C, Sun P, Xie J, et al. Two-dimensional IrO2/MnO2 enabling conformal growth of amorphous Li2O2 for high-performance Li-O2 batteries. Energy Stor Mater 2017;9:206-13.
225. Zhang P, He M, Xu S, Yan X. The controlled growth of porous δ-MnO2 nanosheets on carbon fibers as a bi-functional catalyst for rechargeable lithium-oxygen batteries. J Mater Chem A 2015;3:10811-8.
226. Zhang J, Li P, Wang Z, et al. Three-dimensional graphene-Co3O4 cathodes for rechargeable Li-O2 batteries. J Mater Chem A 2015;3:1504-10.
227. Song K, Cho E, Kang Y. Morphology and active-site engineering for stable round-trip efficiency Li-O2 batteries: a search for the most active catalytic site in Co3O4. ACS Catal 2015;5:5116-22.
228. Liu Q, Xu J, Chang Z, Zhang X. Direct electrodeposition of cobalt oxide nanosheets on carbon paper as free-standing cathode for Li-O2 battery. J Mater Chem A 2014;2:6081-5.
229. Hu X, Cheng F, Zhang N, Han X, Chen J. Nanocomposite of Fe2O3@C@MnO2 as an efficient cathode catalyst for rechargeable lithium-oxygen batteries. Small 2015;11:5545-50.
230. Hong M, Choi HC, Byon HR. Nanoporous NiO plates with a unique role for promoted oxidation of carbonate and carboxylate species in the Li-O2 battery. Chem Mater 2015;27:2234-41.
231. Zhang Y, Hu M, Yuan M, et al. Ordered two-dimensional porous Co3O4 nanosheets as electrocatalysts for rechargeable Li-O2 batteries. Nano Res 2019;12:299-302.
232. Zheng Y, Gao R, Zheng L, Sun L, Hu Z, Liu X. Ultrathin Co3O4 nanosheets with edge-enriched {111} planes as efficient catalysts for lithium-oxygen batteries. ACS Catal 2019;9:3773-82.
233. Li JH, Yu YX. How Do oxygen vacancies influence the catalytic performance of two-dimensional Nb2O5 in lithium- and sodium-oxygen batteries? ChemSusChem 2021;14:5488-98.
234. Long Y, Zhang Z, Zhao L, et al. Bucket effect on high-performance Li-O2 batteries based on P-doped 3D NiO microspheres with conformal growth of discharge products. J Mater Chem A 2022;10:24538-51.
235. Wang G, Zhang S, Qian R, Wen Z. Atomic-Thick TiO2(B) nanosheets decorated with ultrafine Co3O4 nanocrystals as a highly efficient catalyst for lithium-oxygen battery. ACS Appl Mater Interfaces 2018;10:41398-406.
236. Wang H, Fan B, Luo Z, Wu Q, Zhou X, Wang F. A unique hierarchical structure: NiCo2O4 nanowire decorated NiO nanosheets as a carbon-free cathode for Li-O2 battery. Catal Sci Technol 2021;11:7632-9.
237. Song K, Ai W, Zhang Y, et al. Three-dimensional self-supported CuCo2O4 nanowires@NiO nanosheets core/shell arrays as an oxygen electrode catalyst for Li-O2 batteries. J Mater Chem A 2021;9:3007-17.
238. Liu G, Zhang L, Wang S, Ding L, Wang H. Hierarchical NiCo2O4 nanosheets on carbon nanofiber films for high energy density and long-life Li-O2 batteries. J Mater Chem A 2017;5:14530-6.
239. Suntivich J, Gasteiger HA, Yabuuchi N, Nakanishi H, Goodenough JB, Shao-Horn Y. Design principles for oxygen-reduction activity on perovskite oxide catalysts for fuel cells and metal-air batteries. Nat Chem 2011;3:546-50.
240. Kim C, Gwon O, Jeon I, et al. Cloud-like graphene nanoplatelets on Nd0.5Sr0.5CoO3-δ nanorods as an efficient bifunctional electrocatalyst for hybrid Li-air batteries. J Mater Chem A 2016;4:2122-7.
241. Zhou Y, Yan D, Gu Q, et al. Implanting cation vacancies in Ni-Fe LDHs for efficient oxygen evolution reactions of lithium-oxygen batteries. Appl Catal B 2021;285:119792.
242. Sun Z, Lin L, Yuan M, et al. Two-dimensional β-cobalt hydroxide phase transition exfoliated to atom layers as efficient catalyst for lithium-oxygen batteries. Electrochim Acta 2018;281:420-8.
243. Zhu J, Metzger M, Antonietti M, Fellinger TP. Vertically aligned two-dimensional graphene-metal hydroxide hybrid arrays for Li-O2 batteries. ACS Appl Mater Interfaces 2016;8:26041-50.
244. Wang X, Wang Q, Hou X, et al. Facile fabrication of two-dimensional reduced graphene oxide/CoAl-layered double hydroxides nanocomposites for lithium-oxygen battery with improved electrochemical performance. J Alloys Compd 2018;744:196-203.
245. Lu X, Sakai N, Tang D, et al. CoNiFe Layered Double Hydroxide/RuO2.1 nanosheet superlattice as carbon-free electrocatalysts for water splitting and Li-O2 batteries. ACS Appl Mater Interfaces 2020;12:33083-93.
246. Ding S, Liu S, Li J, Wu L, Ma ZF, Yuan X. Multifunctional catalyst CuS for nonaqueous rechargeable lithium-oxygen batteries. ACS Appl Mater Interfaces 2021;13:50065-75.
247. Hou Z, Shu C, Hei P, et al. Configuration of gradient-porous ultrathin FeCo2S4 nanosheets vertically aligned on Ni foam as a noncarbonaceous freestanding oxygen electrode for lithium-oxygen batteries. Nanoscale 2020;12:1864-74.
248. Sadighi Z, Liu J, Ciucci F, Kim JK. Mesoporous MnCo2S4 nanosheet arrays as an efficient catalyst for Li-O2 batteries. Nanoscale 2018;10:15588-99.
249. Zhang P, Hui X, Wang H, Gao X, Yin L. Porous hollow ZnCo2S4 nanosheet arrays derived from metal-organic framework as efficient cathode for lithium oxygen batteries. J Alloys Compd 2021;860:157656.
250. Zhang G, Li G, Wang J, et al. 2D SnSe cathode catalyst featuring an efficient facet-dependent selective Li2O2 growth/decomposition for Li-oxygen batteries. Adv Energy Mater 2022;12:2103910.
251. Guo L, Tan L, Xu A, et al. Highly efficient two-dimensional Ag2Te cathode catalyst featuring a layer structure derived catalytic anisotropy in lithium-oxygen batteries. Energy Stor Mater 2022;50:96-104.
252. Ran Z, Shu C, Hou Z, et al. Phosphorus vacancies enriched Ni2P nanosheets as efficient electrocatalyst for high-performance Li-O2 batteries. Electrochim Acta 2020;337:135795.
253. Wei M, Luo Y, Jin C, et al. MoP nanoflakes as efficient electrocatalysts for rechargeable Li-O2 batteries. ACS Appl Energy Mater 2018;1:331-5.
254. Ma Z, Yuan X, Zhang Z, et al. Novel flower-like nickel sulfide as an efficient electrocatalyst for non-aqueous lithium-air batteries. Sci Rep 2015;5:18199.
255. Hou Z, Long J, Shu C, Liang R, Li J, Liao X. Two-dimensional spinel CuCo2S4 nanosheets as high efficiency cathode catalyst for lithium-oxygen batteries. J Alloys Compd 2019;798:560-7.
256. He B, Li G, Li J, et al. MoSe2@CNT core-shell nanostructures as grain promoters featuring a direct Li2O2 formation/decomposition catalytic capability in lithium-oxygen batteries. Adv Energy Mater 2021;11:2003263.
257. Hu A, Shu C, Qiu X, Li M, Zheng R, Long J. Improved cyclability of lithium-oxygen batteries by synergistic catalytic effects of two-dimensional MoS2 nanosheets anchored on hollow carbon Spheres. ACS Sustain Chem Eng 2019;7:6929-38.
258. Lai Y, Chen W, Zhang Z, Gan Y, Yang X, Li J. Two-dimensional graphene-like MoSe 2 nanosheets anchored on hollow carbon nanofibers as a cathode catalyst for rechargeable Li-O2 batteries. RSC Adv 2016;6:19843-7.
259. Sun Z, He J, Yuan M, et al. Li+-clipping for edge S-vacancy MoS2 quantum dots as an efficient bifunctional electrocatalyst enabling discharge growth of amorphous Li2O2 film. Nano Energy 2019;65:103996.
260. Sadighi Z, Liu J, Zhao L, Ciucci F, Kim JK. Metallic MoS2 nanosheets: multifunctional electrocatalyst for the ORR, OER and Li-O2 batteries. Nanoscale 2018;10:22549-59.
261. Wang J, Gao R, Zheng L, et al. CoO/CoP Heterostructured nanosheets with an O-P interpenetrated interface as a bifunctional electrocatalyst for Na-O2 battery. ACS Catal 2018;8:8953-60.
262. Yan Y, Ran Z, Zeng T, et al. Interfacial electron redistribution of hydrangea-like NiO@Ni2P heterogeneous microspheres with dual-phase synergy for high-performance lithium-oxygen battery. Small 2022;18:e2106707.
263. Yang Y, Chen J, Gao Q, Feng Y, Xing F, Yao M. First-principle study on catalytic activity of functionalized Ti3C2 MXene as cathode catalyst for Li-O2 batteries. Current Appl Phys 2022;34:24-8.
264. Jiang Y, Tian M, Wang H, et al. Mildly oxidized MXene (Ti3C2, Nb2C, and V2C) electrocatalyst via a generic strategy enables longevous Li-O2 battery under a high rate. ACS Nano 2021;15:19640-50.
265. Li G, Li N, Peng S, et al. Highly efficient Nb2C MXene cathode catalyst with uniform O-terminated surface for lithium-oxygen batteries. Adv Energy Mater 2021;11:2002721.
266. Li J, Han K, Huang J, et al. Polarized nucleation and efficient decomposition of Li2O2 for Ti2C MXene cathode catalyst under a mixed surface condition in lithium-oxygen batteries. Energy Stor Mater 2021;35:669-78.
267. Zhao D, Wang P, Di H, Zhang P, Hui X, Yin L. Single semi-metallic selenium atoms on Ti3C2 MXene nanosheets as excellent cathode for lithium-oxygen batteries. Adv Funct Mater 2021;31:2010544.
268. Zheng R, Shu C, Hou Z, et al. In Situ Fabricating oxygen vacancy-rich TiO2 nanoparticles via utilizing thermodynamically metastable Ti atoms on Ti3C2Tx MXene nanosheet surface to boost electrocatalytic activity for high-performance Li-O2 batteries. ACS Appl Mater Interfaces 2019;11:46696-704.
269. Zheng R, Shu C, Chen X, et al. Unique intermediate adsorption enabled by anion vacancies in metal sulfide embedded MXene nanosheets overcoming kinetic barriers of oxygen electrode reactions in lithium-oxygen batteries. Energy Stor Mater 2021;40:41-50.
270. Lv Q, Zhu Z, Ni Y, et al. Atomic ruthenium-riveted metal-organic framework with tunable d-band modulates oxygen redox for lithium-oxygen batteries. J Am Chem Soc 2022;144:23239-46.
271. Lv Q, Zhu Z, Ni Y, Geng J, Li F. Spin-state manipulation of two-dimensional metal-organic framework with enhanced metal-oxygen covalency for lithium-oxygen batteries. Angew Chem Int Ed 2022;61:e202114293.
272. Luo WB, Chou SL, Wang JZ, Zhai YC, Liu HK. A metal-free, free-standing, macroporous graphene@g-C₃N₄ composite air electrode for high-energy lithium oxygen batteries. Small 2015;11:2817-24.
273. Yi J, Liao K, Zhang C, Zhang T, Li F, Zhou H. Facile in situ preparation of graphitic-C3N4@carbon paper as an efficient metal-free cathode for nonaqueous Li-O2 battery. ACS Appl Mater Interfaces 2015;7:10823-7.
274. Mehri M, Mousavi-khoshdel S, Molaei M. First-principle calculations study of pristine, S-, O-, and P-doped g-C3N4 as ORR catalysts for Li-O2 batteries. Chem Phys Lett 2021;775:138614.
275. Hang Y, Zhang C, Luo X, et al. α-MnO2 nanorods supported on porous graphitic carbon nitride as efficient electrocatalysts for lithium-air batteries. J Power Sources 2018;392:15-22.
276. Wu Y, Wang T, Zhang Y, et al. Electrocatalytic performances of g-C3N4-LaNiO3 composite as bi-functional catalysts for lithium-oxygen batteries. Sci Rep 2016;6:24314.
Cite This Article
Export citation file: BibTeX | RIS
OAE Style
Pei C, Zhang D, Kim J, Yu X, Sim U, Park HS, Kim JK. Bifunctional 2D structured catalysts for air electrodes in rechargeable metal-air batteries. Energy Mater 2024;4:400003. http://dx.doi.org/10.20517/energymater.2023.66
AMA Style
Pei C, Zhang D, Kim J, Yu X, Sim U, Park HS, Kim JK. Bifunctional 2D structured catalysts for air electrodes in rechargeable metal-air batteries. Energy Materials. 2024; 4(1): 400003. http://dx.doi.org/10.20517/energymater.2023.66
Chicago/Turabian Style
Pei, Chengang, Dong Zhang, Jaekyum Kim, Xu Yu, Uk Sim, Ho Seok Park, Jung Kyu Kim. 2024. "Bifunctional 2D structured catalysts for air electrodes in rechargeable metal-air batteries" Energy Materials. 4, no.1: 400003. http://dx.doi.org/10.20517/energymater.2023.66
ACS Style
Pei, C.; Zhang D.; Kim J.; Yu X.; Sim U.; Park HS.; Kim JK. Bifunctional 2D structured catalysts for air electrodes in rechargeable metal-air batteries. Energy Mater. 2024, 4, 400003. http://dx.doi.org/10.20517/energymater.2023.66
About This Article
Special Issue
Copyright
Data & Comments
Data
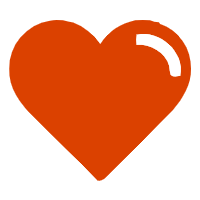

Comments
Comments must be written in English. Spam, offensive content, impersonation, and private information will not be permitted. If any comment is reported and identified as inappropriate content by OAE staff, the comment will be removed without notice. If you have any queries or need any help, please contact us at support@oaepublish.com.