Metal- and binder-free dual-ion battery based on green synthetic nano-embroidered spherical organic anode and pure ionic liquid electrolyte
Abstract
Dual-ion batteries (DIBs) have attracted extensive attention and investigations due to their inherent wide operating voltage and environmental friendliness. Nevertheless, the vast majority of DIBs employ metal-based anode active materials or electrolytes, which are relatively costly and unsustainable. Moreover, the utilization of binders and current collectors in the preparation of cathodes and anodes reduces the energy density to a certain extent, which weakens the advantages of DIBs. Here, we synthesized three types of binder-free nano-embroidered spherical polyimide anode materials composed entirely of renewable elements, paired with pure ionic liquid electrolyte without metal elements and flexible self-supporting independent graphite paper cathode without current collector, to construct a class of totally metal and binder-free DIBs. It significantly improves specific discharge capacity, energy density, cyclic stability, and fast charging performance while remarkably reducing costs and self-discharge rate. Additionally, we overcame the drawbacks of conventional synthesis methods and innovatively prepared nanoscale polyimide materials by a green and facile hydrothermal method, which effectively minimizes synthesis costs and avoids risks. This novel battery system design strategy will promote the advancement of low-cost, high-performance DIBs and could be a promising candidate for large-scale energy storage applications.
Keywords
INTRODUCTION
The high energy density, long cyclic life, and no memory effect of lithium-ion batteries (LIBs) enable them to occupy a major share of the electrochemical energy storage (EES) market since their commercialization and are widely used in portable electronic devices and electric vehicles[1-4]. However, the scarcity and uneven distribution of metal resources, such as lithium and cobalt, drive up the manufacturing and usage costs of LIBs year by year, rendering it difficult to achieve large-scale grid-level energy storage applications. Against such a background, there is an urgent requirement to develop novel battery systems to meet the imminent demand for green and sustainable energy storage programs[5-8]. Different from LIBs, dual-ion batteries (DIBs) allow the simultaneous participation of anions and cations in the electrochemical reaction during charging and discharging, and electrolyte is the singular source of active ions[9-12]. Additionally, inexpensive graphite can be employed to avoid the use of expensive transition metal-based materials as the cathode. This unique reaction mechanism endows DIBs with high cost-effectiveness, suitable operating voltage, and environmental friendliness, which have continuously attracted great interest and research enthusiasm from researchers in recent years[13-15].
Currently, the anode of DIBs is mainly based on inorganic materials such as carbonaceous materials[16-18], alloy-based materials[19-21] and transition metal-based compounds[22-24], which are based on intercalation, alloying, and transformation reactions to accomplish the storage of active ions, respectively. Unfortunately, DIBs based on these electrodes usually undergo structural exfoliation and volume expansion during cycling, causing poor stability, low specific discharge capacity (SDC), and a reduced initial Coulomb efficiency (ICE). In particular, this is not in line with the development concept of "green and sustainable batteries"[25,26]. The key technology for green, sustainable DIBs is the exploration of organic anode materials composed entirely of renewable elements from nature. Organic materials are considered as excellent candidates for the next generation of green and sustainable EES due to their inherent merits of being metal-free and widely available, eco-friendly, cost-effective, and structurally well-designed[27-33]. However, an understanding of their application in DIBs is still in its infancy. Several organic materials have been reported, such as polycyclic aromatic hydrocarbon[34,35], nitrogen/sulfur-containing organics[36,37], carbonyl compounds[38,39], and so on. Organic carbonyl compounds are of great research fascination owing to their simplified synthetic route, unique multi-electron reaction, and structural diversity[40]. Unfortunately, small molecule carbonyl compounds inevitably dissolve in organic electrolytes, leading to terrible cyclic stability and accelerated capacity degradation, limiting their application in large-scale energy storage. Numerous attempts have been made by investigators to avoid dissolution and achieve excellent electrochemical performance. Due to their composition of organic cations and inorganic anions, ionic liquids have a broad electrochemical window. They are kinetically stable, free of solvent molecules, and non-flammable. Therefore, a feasible alternative is to adopt ionic liquids instead of traditional organic solvents[41-43]. Particularly, ionic liquids operate both as solvents and as active ions participating in electrochemical reactions, not only effectively preventing dissolution but also avoiding the use of electrolyte metal salts. Another feasible approach is to design organic polymers with stable inactive backbones and highly redox-active carbonyl functional groups such as polyimide (PI) electrode materials[44]. Additionally, employing nano-engineering design strategies can further increase the active sites of electrode materials and facilitate the transport of active ions and electrolyte penetration, which is beneficial for improving the SDC and electrode reaction kinetics[45,46]. Nevertheless, the application of PI as anodes for DIBs has rarely been reported. One of the main challenges is the dilemma of synthesis, followed by a long (> 8 h) polycondensation reaction at high temperatures (> 200 °C) under a catalyst and N2 atmosphere, and the final product also needs to be baked in a tube furnace at high temperatures (> 300 °C) for more than 8 h to remove the solvent[47,48]. As a consequence, the whole procedure is time-consuming and costly, with certain technical challenges and safety risks. In this regard, it is essential to explore a cost-effective, energy-saving, green, and safe synthesis path to obtain comparable or even superior performance.
Herein, after continuous trial and exploration, we have developed a simplified one-step green synthesis strategy, and three types of PI nano-anode materials can be obtained directly by hydrothermal reactions at 160 °C for 6 h. Besides, the straightforward adoption of independent graphite paper (GP) with self-supporting capability as the cathode and current collector, and the anode is binder-free, which greatly improves the discharge capacity and energy density. Combined with a pure ionic liquid electrolyte, a dual-ion full battery system without metal elements and binder is constructed. The reaction principle of the electrodes and the working mechanism of the battery are investigated through a series of physical and chemical characterizations. Such innovative DIBs with outstanding electrochemical performance furnish insight into the establishment of greener and sustainable EES systems and are expected to be applied in large-scale energy storage.
EXPERIMENTAL
Materials
Pyromellitic anhydride, o/m/p-phenylenediamine (o/m/p-PDA), N-methylpyrrolidone (NMP), and GP were purchased from Macklin. Glass fiber diaphragm (GF/A), conductive agent (Super P), carbon-coated aluminum foil, and CR2025 type cell shells were purchased from Clorod. N-butyl-N-methylpyrrolidinium bis(trifluoromethanesulfonyl)imide ionic liquid was purchased from Lanzhou Institute of Chemical Physics, Chinese Academy of Sciences. Unless otherwise stated, no further treatment was required for all materials.
Synthesis of o-PDI, m-PDI, and p-PDI
Firstly, homophthalic anhydride and o/m/p-PDA are added sequentially to 110 mL of deionized water in a 1:3 molar ratio and stirred overnight at room temperature on a magnetic stirrer, then poured into a reaction kettle equipped with 250 mL PTFE liner and finally reacted hydrothermally in an oven at 160 °C for 6 h. After cooling to room temperature, the reaction was filtered with water, methanol, and ethanol successively and dried in a freeze dryer for 48 h. The as-prepared samples were named as o-PDI, m-PDI, and p-PDI.
Materials characterizations
Scanning electron microscopy (SEM) images and energy-dispersive X-ray spectroscopy (EDX) spectra and corresponding mappings were acquired on a scanning electron microscope (Merlin, ZEISS, Germany).
Battery assembly and measurements
In order to evaluate the electrochemical performance of the dual ion battery, the full battery was assembled by using CR-2025 type button cells in a super glove box (H2O < 0.01 ppm, O2 < 0.01 ppm), in which the cathode is directly employed with independent GP with self-supporting capability. As for the preparation of the anode, o/m/p-PDI and Super P are mixed in the ratio of 6:4 and added to a weighing bottle containing NMP without any binder added. Magnetic stirring was done overnight to form a homogeneous slurry, which was later coated on top of the carbon-coated aluminum foil with a coating thickness of 100 μm. The coating is then transferred to a vacuum drying oven at 100 °C for 12 h. Both the cathode and anode are cut into 14 mm diameter discs and placed in a glove box for use. The mass loading of the anode is
RESULTS AND DISCUSSION
Physical characterizations
As shown in Supplementary Schematic 1, three types of PI materials (o-PDI, m-PDI, and p-PDI) were synthesized by using three phenylenediamine isomers (o/m/p-PDA) and pyromellitic dianhydride (PMDA) through a green hydrothermal polycondensation reaction and freeze-drying. Figure 1A-C presents the SEM of the as-prepared o-PDI, m-PDI, and p-PDI, respectively, which all exhibit an embroidered spherical morphology. The elemental mapping in Figure 1D and Supplementary Figures 1 and 2 shows that the C, N, and O elements are uniformly distributed. Specifically, each hydrangea is composed of nanoscale flakes with thicknesses between 10~20 nm and displays a three-dimensional porous network interleaved structure. This distinctive nano-morphology configuration facilitates adequate electrolyte infiltration, diffusion, and storage of active ions, thus improving the ion transport kinetics, rate capability, and discharge capacity. Besides,
Figure 1. Characterizations of the o-PDI, m-PDI, and p-PDI. (A-C) SEM. (D) Elemental analysis. (E) FT-IR. (F) XRD. (G-J) XPS. (K) TGA. (L and M) N2 adsorption/desorption curves and pore size distributions.
The structural stability of the materials was investigated via the dissolution of the electrode sheets in electrolyte and TGA, respectively. The Pyr14TFSI ionic liquid electrolyte placed with PMDA, o-PDI, m-PDI, and p-PDI electrode sheets, respectively, showed no variations with time migration and remained clear and transparent even after one month [Supplementary Figure 6], indicating that o-PDI/m-PDI/p-PDI exhibits excellent stability in this ionic liquid. This is supported by the TGA curves in Figure 1K, where the three phenylenediamine isomers decomposed at ~150 °C with significant mass loss, and the PMDA monomer also decomposed at around ~250 °C with poor stability. On the contrary, the stability of o/m/p-PDI increased noticeably, with the onset decomposition temperature of o-PDI and p-PDI reaching up to
Electrochemical performance and kinetics of o-PDI, m-PDI, and p-PDI
A Pyr14TFSI ionic liquid electrolyte-based button-type battery was used to probe the active ion storage capacity and reaction kinetics of the electrodes. Supplementary Figure 7 shows the two-electrode cyclic voltametric (CV) curves based on PMDA electrodes at different cycles scanned from the open circuit voltage in the negative direction at a rate of 1 mV/s. No redox peaks appear, indicating that no electrochemical redox reaction occurs and the PMDA electrode cannot store active ions[61,62]. Interestingly, when replaced with the o-PDI/m-PDI/p-PDI electrode, two pairs of sharp redox peaks emerge, as shown in Figure 2A-C, respectively, and all the CV curves overlap, indicating excellent cycling reversibility and stability. Figure 2D and Supplementary Figure 8A-C depict the GCD curves based on PMDA, o-PDI, p-PDI, and m-PDI anodes, respectively. The full battery system, on the basis of PMDA anodes, exhibits two inclined curves without charge/discharge plateaus [Supplementary Figure 8A]. In contrast, the systems based on m-PDI, o-PDI, and p-PDI anodes all display typical active ion insertion/de-insertion behavior with two pairs of symmetrical charge/discharge plateaus [Figure 2D and Supplementary Figure 8B and C], respectively, in accordance with the CV curves. Similar to the GCD curves, the dQ/dV curves in Supplementary Figure 9 indicate that the charging and discharging processes of o-PDI, p-PDI, and m-PDI are divided into two regions except for PMDA, which represent the various stages of insertion and de-insertion, respectively[63-65]. The electrochemical storage behavior and reaction kinetics of o-PDI, m-PDI, and p-PDI were further analyzed by CV curves at different scan rates (0.2/0.4/0.6/0.8/1 mV/s), and the results are summarized in Figure 2E and Supplementary Figure 10A and B. All of the CV curves manifest an analogous silhouette and well-defined redox peaks, which indicate a stable electrochemical performance[23,66]. The voltammetric response of the electrode at various scan rates can be quantified based on the power-law relationship
Figure 2. Electrochemical performance and kinetic characterizations of o-PDI, m-PDI, and p-PDI electrodes. (A-C) CV tests at 1 mV/s. (D) GCD curves. (E) CV curves at various scan rates and (F) The corresponding b values derived from i = avb. (G) Pseudocapacitance ratio of m-PDI at 1 mV/s. (H) Contribution of pseudocapacitance at different scan rates. (I) Linear relationship between i and the square root of v.
Quantitatively, the capacitive contribution is calculated based on Dunn’s method: i = k1v + k2v1/2, where k1 and k2 are constants, and k1v and k2v1/2 represent the pseudocapacitance contribution and the diffusion contribution, respectively. Consequently, the pseudocapacitance contributions of o-PDI, m-PDI, and p-PDI electrodes at 1 mV/s are 0.69, 0.95, and 0.89 (the red-filled part in Figure 2G and
Electrochemical performance of o//G-DIB, m//G-DIB, p//G-DIB
The proof-of-concept full batteries based on o-PDI/m-PDI/p-PDI anodes, GP cathodes, and Pyr14TFSI ionic liquid electrolytes were constructed respectively to further investigate the electrochemical performance for various systems (abbreviated as o//G-DIB, m//G-DIB, p//G-DIB). In particular, GP is a type of conductive carbon material featuring self-supporting flexibility capability without current collectors
Figure 3. Electrochemical performances of o//G-DIB, m//G-DIB, and p//G-DIB. (A-C) GCD curves at different rates. (D) dQ/dV curves of m//G-DIB. (E) Rate performance. (F) EIS and its fitting diagram. (G) Cyclic performance at 2 C. (H) The medium discharge voltage during cycling. (I) The long-term cyclic performance under 10 C.
The cyclic performance of o//G-DIB, m//G-DIB, and p//G-DIB at 2 C is compared, as shown in Figure 3G, where the reversible initial specific discharge capacities of o//G-DIB and p//G-DIB are 94 and 104 mA h g-1, respectively, and the capacity retentions after 850 cycles are 99% and 99.5%, respectively. Apparently, the
A low self-discharge rate is an essential performance parameter for full batteries to achieve practical applications, and unfortunately, DIBs are often criticized for exhibiting a comparatively high self-discharge rate. On this basis, the self-discharge performance of o//G-DIB, m//G-DIB, and p//G-DIB was tested separately by fully charging the batteries first and then completely discharging them after 10 h of resting. The results are presented in Figure 4A-F and Supplementary Figure 22, according to the self-discharge rate calculation formula: S = (C - Cr)/CT*100%, where S denotes the self-discharge rate, C and Cr are the SDCs without and after resting, respectively, and T refers to the resting time[23,80]. It can be calculated that the self-discharge rates of o//G-DIB, m//G-DIB, and p//G-DIB are 0.00817, 0.00371 and 0.00603 h-1, respectively, which are significantly lower than the currently reported battery systems, as shown in
Working mechanism and reaction principle
Taking the m//G-DIB system as a typical representative, the unique active ion storage mechanism of cathodes/anodes and the variations of chemical bonds during charging and discharging were investigated by FT-IR, Raman, and XPS. Figure 5A and Supplementary Figure 23 illustrate the FT-IR characterization of the anode under different charging and discharging states. The symmetric and asymmetric stretching vibration peaks of C=O located near 1,742 and 1,769 cm-1 basically disappear after being completely charged [Figure 5A], and the characteristic peak of C-O appears near 1,270 cm-1 [Supplementary Figure 23]. Meanwhile, it is evident from the fitted high-resolution N1s spectra that N-S and N-F characteristic peaks appeared at 402 and 399 eV, respectively, after completely charged [Figure 5B and
Figure 5. The investigations of energy storage mechanism based on cathodes and anodes. (A) FT-IR and (B) the fitted high-resolution
From the above characterizations, combined with the CV, dQ/dV, and GCD curves in Figure 2, it can be concluded that the working mechanism of the full battery is depicted in Figure 5E. The electrode reactions involved are shown in (1) to (3): during charging, the active cations and anions in the electrolyte move toward the cathode and anode, respectively, and participate in the electrochemical reactions. Conversely, during the discharge process, the cations and anions return to the electrolyte from the cathode and anode, respectively. Additionally, as shown in Figure 5F, only one coin battery is able to light the LED and successfully charge the smartwatch, indicating a great prospect for practical applications.
Anode: x(o/m/p - PDI)n+ Pyr14+ + 2nxe- ↔ (o/m/p - PDI)nx(Pyr14)2nx
Cathode: yC + 2nxTFSI- ↔ Cy (TFSI)2nx+ 2nxe-
Overall: x(o/m/p-PDI)n+ 2nxPyr14+ + yC + 2nxTFSI- ↔ (o/m/p - PDI)nx(Pyr14)2nx+ Cy(TFSI)2nx
Such excellent electrochemical performance is greatly dependent on the superior structural stability of the cathode and anode. To appreciate this, a combination of XRD, Raman, and FT-IR techniques was employed to characterize the electrodes during the charge and discharge process. For the GP cathode, as depicted in Figure 6A and B, the signal of the 002 characteristic peak located at 26.5° decreases gradually during the charging process and drops to a minimum after being completely charged, implying the formation of graphite intercalation compounds (GICs) accompanied by a reduction in crystallinity due to the intercalation of TFSI-[67,75,86]. It can be corroborated by Raman that during the charging process, the graphite layers adjacent to the intercalation layers become highly charged owing to the formation of GICs, and the G peak located at 1,580 cm-1 undergoes a blue shift accompanied by a weakening of the signal
Figure 6. XRD, Raman, and FT-IR characterizations at different charge and discharge states. (A, B, F) XRD and (C, D, G) Raman and FT-IR spectrums of the cathode and anode based on the selected stages. (E) The IG/ID values based on different charging and discharging stages. (H) Electrochemical performance comparison of recently reported work in DIBs.
CONCLUSIONS
In summary, three types of PI anode materials with distinctive nano-morphologies, o-PDI, m-PDI, and p-PDI, could be synthesized on a large scale by an innovative green and mild hydrothermal strategy, which breaks through the technical drawbacks of the traditional synthesis of PIs. Through a combination of a series of physicochemical characterizations and electrochemical measurements, it is proved that m-PDI shows the most excellent performance due to the homogeneous and well-dispersed lamellar structure, the maximum specific surface area and pore size, the optimum ion diffusion coefficient, and the highest pseudocapacitance ratio. Matching with the GP cathode with self-supporting flexibility and Pyr14TFSI pure ionic liquid electrolytes, the binder and metal-free o//G-DIB, m//G-DIB, and p//G-DIB full battery systems are constructed, and they exhibit outstanding electrochemical performances: (1) high specific discharge capacities of 144, 222 and 168 mA h g-1 at 0.2 C, respectively, and energy densities of 409.8 and
DECLARATIONS
Acknowledgments
The authors would like to acknowledge the financial support from the National Natural Science Foundation of China (No. 22075089) and the Fundamental and Applied Fundamental Research Project of Zhuhai City (No. 22017003200023).
Authors’ contributions
Prepared the o/m/p-PDI, assembled the dual-ion batteries, and performed most of the electrochemical experiments and physicochemical characterizations: Wu H
Supervised the project: Fang Y, Yuan W
Analyzed the data and co-wrote and discussed the whole paper: Wu H, Luo S, Zheng W, Li L, Fang Y,
Availability of data and materials
The data are made available upon request to authors.
Financial support and sponsorship
This work was financially supported by the National Natural Science Foundation of China (No. 22075089) and the Fundamental and Applied Fundamental Research Project of Zhuhai City (No. 22017003200023). The authors would like to thank Jian-ming Liu from the Shiyanjia Lab (www.shiyanjia.com) for the support of SEM, XRD, and Raman testing.
Conflicts of interest
All authors declared that there are no conflicts of interest.
Ethical approval and consent to participate
Not applicable.
Consent for publication
Not applicable.
Copyright
© The Author(s) 2024.
Supplementary Materials
REFERENCES
1. Li J, Fleetwood J, Hawley WB, Kays W. From materials to cell: state-of-the-art and prospective technologies for lithium-ion battery electrode processing. Chem Rev 2022;122:903-56.
2. Zhang H, Wang L, He X. Trends in a study on thermal runaway mechanism of lithium-ion battery with LiNixMnyCo1-x-yO2 cathode materials. Battery Energy 2022;1:20210011.
3. Neumann J, Petranikova M, Meeus M, et al. Recycling of lithium-ion batteries - current state of the art, circular economy, and next generation recycling. Adv Energy Mater 2022;12:2102917.
4. Wang E, Xiao D, Wu T, et al. Stabilizing oxygen by high-valance element doping for high-performance Li-rich layered oxides. Battery Energy 2023;2:20220030.
5. Du K, Ang EH, Wu X, Liu Y. Progresses in sustainable recycling technology of spent lithium-ion batteries. Energy Environ Mater 2022;5:1012-36.
6. Gao C, Jiang Z, Qi S, et al. Metal-organic framework glass anode with an exceptional cycling-induced capacity enhancement for lithium-ion batteries. Adv Mater 2022;34:e2110048.
7. Liu Y, Russo PA, Montoro LA, Pinna N. Recent developments in Nb-based oxides with crystallographic shear structures as anode materials for high-rate lithium-ion energy storage. Battery Energy 2023;2:20220037.
8. Lee MJ, Han J, Lee K, et al. Elastomeric electrolytes for high-energy solid-state lithium batteries. Nature 2022;601:217-22.
9. Ji B, Zhang F, Song X, Tang Y. A novel potassium-ion-based dual-ion battery. Adv Mater 2017;29:201700519.
10. Wu X, Xu Y, Zhang C, et al. Reverse dual-ion battery via a ZnCl2 water-in-salt electrolyte. J Am Chem Soc 2019;141:6338-44.
11. Zhang X, Tang Y, Zhang F, Lee CS. A novel aluminum-graphite dual-ion battery. Adv Energy Mater 2016;6:1502588.
12. Li Y, Wang B. High rate and ultralong cyclelife fiber-shaped sodium dual-ion battery based on bismuth anodes and polytriphenylamine cathodes. Battery Energy 2023;2:20220035.
13. Wei B, Hong Y, Tang W, et al. Design of bipolar polymer electrodes for symmetric Li-dual-ion batteries. Chem Eng J 2023;451:138773.
14. Das S, Manna SS, Pathak B. Recent advancements in devising computational strategies for dual-ion batteries. ChemSusChem 2023;16:e202201405.
15. Yu M, Liu H, Xiao K, Xie B, Han Z, Wang DW. Redox-mediated proton transport of two-dimensional polyaniline-based nanochannels for fast capacitive performance. Battery Energy 2022;1:20210004.
16. Feng Y, Chen S, Wang J, Lu B. Carbon foam with microporous structure for high performance symmetric potassium dual-ion capacitor. J Energy Chem 2020;43:129-38.
17. Rothermel S, Meister P, Schmuelling G, et al. Dual-graphite cells based on the reversible intercalation of bis(trifluoromethanesulfonyl)imide anions from an ionic liquid electrolyte. Energy Environ Sci 2014;7:3412-23.
18. Wang G, Yu M, Feng X. Carbon materials for ion-intercalation involved rechargeable battery technologies. Chem Soc Rev 2021;50:2388-443.
19. Ou X, Li J, Tong X, Zhang G, Tang Y. Highly concentrated and nonflammable electrolyte for high energy density K-based dual-ion battery. ACS Appl Energy Mater 2020;3:10202-8.
20. Sheng M, Zhang F, Ji B, Tong X, Tang Y. A novel tin-graphite dual-ion battery based on sodium-ion electrolyte with high energy density. Adv Energy Mater 2017;7:1601963.
21. Bizuneh GG, Adam AMM, Ma J. Progress on carbon for electrochemical capacitors. Battery Energy 2023;2:20220021.
22. Salunkhe TT, Kadam AN, Kidanu WG, Lee S, Nguyen TL, Kim IT. A diffusion encouraged core-shell heterostructured Co3Sn2@SnO2 anode towards emerging dual ion batteries with high energy density. J Mater Chem A 2021;9:14991-5002.
23. Wu H, Li L, Yuan W. Nano-cubic α-Fe2O3 anode for Li+/Na+ based dual-ion full battery. Chem Eng J 2022;442:136259.
24. Wang X, Qi L, Wang H. Anatase TiO2 as a Na+-storage anode active material for dual-ion batteries. ACS Appl Mater Interfaces 2019;11:30453-9.
25. Kim J, Kim Y, Yoo J, Kwon G, Ko Y, Kang K. Organic batteries for a greener rechargeable world. Nat Rev Mater 2023;8:54-70.
26. Poizot P, Dolhem F. Clean energy new deal for a sustainable world: from non-CO2 generating energy sources to greener electrochemical storage devices. Energy Environ Sci 2011;4:2003-19.
27. Esser B, Dolhem F, Becuwe M, Poizot P, Vlad A, Brandell D. A perspective on organic electrode materials and technologies for next generation batteries. J Power Sources 2021;482:228814.
28. Feng RZ, Zhang X, Murugesan V, et al. Reversible ketone hydrogenation and dehydrogenation for aqueous organic redox flow batteries. Science 2021;372:836-40.
29. Kim J, Kim H, Lee S, et al. A p-n fusion strategy to design bipolar organic materials for high-energy-density symmetric batteries. J Mater Chem A 2021;9:14485-94.
30. Lakraychi AE, Dolhem F, Vlad A, Becuwe M. Organic negative electrode materials for metal-ion and molecular-ion batteries: progress and challenges from a molecular engineering perspective. Adv Energy Mater 2021;11:2101562.
31. Nguyen TP, Easley AD, Kang NA, et al. Polypeptide organic radical batteries. Nature 2021;593:61-6.
33. Zhu W, Huang Y, Jiang B, Xiao R. A metal-free ionic liquid dual-ion battery based on the reversible interaction of 1-butyl-1-methylpyrrolidinium cations with 1,4,5,8-naphthalenetetracarboxylic dianhydride. J Mol Liq 2021;339:116789.
34. Dong S, Li Z, Rodríguez-pérez IA, et al. A novel coronene//Na2Ti3O7 dual-ion battery. Nano Energy 2017;40:233-9.
35. Das S, Bhauriyal P, Pathak B. Polycyclic aromatic hydrocarbons as prospective cathodes for aluminum organic batteries. J Phys Chem C 2021;125:49-57.
36. Li Q, Wang H, Wang HG, Si Z, Li C, Bai J. A self-polymerized nitro-substituted conjugated carbonyl compound as high-performance cathode for lithium-organic batteries. ChemSusChem 2020;13:2449-56.
37. Kolek M, Otteny F, Schmidt P, et al. Ultra-high cycling stability of poly(vinylphenothiazine) as a battery cathode material resulting from π-π interactions. Energy Environ Sci 2017;10:2334-41.
38. Han C, Li H, Li Y, Zhu J, Zhi C. Proton-assisted calcium-ion storage in aromatic organic molecular crystal with coplanar stacked structure. Nat Commun 2021;12:2400.
39. Häupler B, Wild A, Schubert US. Carbonyls: powerful organic materials for secondary batteries. Adv Energy Mater 2015;5:1402034.
40. Yang H, Lee J, Cheong JY, et al. Molecular engineering of carbonyl organic electrodes for rechargeable metal-ion batteries: fundamentals, recent advances, and challenges. Energy Environ Sci 2021;14:4228-67.
41. Shaplov AS, Marcilla R, Mecerreyes D. Recent advances in innovative polymer electrolytes based on poly(ionic liquid)s. Electrochim Acta 2015;175:18-34.
42. Chen Y, Yu D, Liu Z, Xue Z, Mu T. Thermal, chemical, electrochemical, radiolytic and biological stability of ionic liquids and deep eutectic solvents. New J Chem 2022;46:17640-68.
43. Wang A, Yuan W, Fan J, Li L. A dual-graphite battery with pure 1-butyl-1-methylpyrrolidinium bis(trifluoromethylsulfonyl) imide as the electrolyte. Energy Technol 2018;6:2172-8.
44. Song Z, Zhan H, Zhou Y. Polyimides: promising energy-storage materials. Angew Chem Int Ed 2010;49:8444-8.
45. Wu H, Wang K, Meng Y, Lu K, Wei Z. An organic cathode material based on a polyimide/CNT nanocomposite for lithium ion batteries. J Mater Chem A 2013;1:6366-72.
46. Raj M, Mangalaraja RV, Lee G, Contreras D, Zaghib K, Reddy MV. Large π-conjugated condensed perylene-based aromatic polyimide as organic cathode for lithium-ion batteries. ACS Appl Energy Mater 2020;3:6511-24.
47. Jiang B, Kong T, Cai Z, Zhu W, Xiao R. In-situ modification of polyimide anode materials in dual-ion batteries. Electrochim Acta 2022;435:141402.
48. Ba Z, Wang Z, Luo M, et al. Benzoquinone-based polyimide derivatives as high-capacity and stable organic cathodes for lithium-ion batteries. ACS Appl Mater Interfaces 2020;12:807-17.
49. Kapaev RR, Scherbakov AG, Shestakov AF, Stevenson KJ, Troshin PA. m-phenylenediamine as a building block for polyimide battery cathode materials. ACS Appl Energy Mater 2021;4:4465-72.
50. Kim T, Park B, Lee KM, et al. Hydrothermal synthesis of composition- and morphology-tunable polyimide-based microparticles. ACS Macro Lett 2018;7:1480-5.
51. Ham Y, Fritz NJ, Hyun G, et al. 3D periodic polyimide nano-networks for ultrahigh-rate and sustainable energy storage. Energy Environ Sci 2021;14:5894-902.
52. Ryu J, Park B, Kang J, et al. Three-dimensional monolithic organic battery electrodes. ACS Nano 2019;13:14357-67.
53. Chen L, Li W, Wang Y, Wang C, Xia Y. Polyimide as anode electrode material for rechargeable sodium batteries. RSC Adv 2014;4:25369-73.
54. Geng J, Ni Y, Zhu Z, et al. Reversible metal and ligand redox chemistry in two-dimensional iron-organic framework for sustainable lithium-ion batteries. J Am Chem Soc 2023;145:1564-71.
55. Yu Z, Huang L, Sun Z, Cai F, Liang M, Luo Z. Designing anthraquinone-based conjugated microporous polymers with dual-ion storage behavior towards high-performance lithium-organic batteries. J Power Sources 2022;550:232149.
56. Xiu Y, Mauri A, Dinda S, et al. Anion storage chemistry of organic cathodes for high-energy and high-power density divalent metal batteries. Angew Chem Int Ed 2023;62:e202212339.
57. Zhang Y, Nie P, Xu C, et al. High energy aqueous sodium-ion capacitor enabled by polyimide electrode and high-concentrated electrolyte. Electrochim Acta 2018;268:512-9.
58. Wu H, Ye Z, Zhu J, Luo S, Li L, Yuan W. High discharge capacity and ultra-fast-charging sodium dual-ion battery based on insoluble organic polymer anode and concentrated electrolyte. ACS Appl Mater Interfaces 2022;14:49774-84.
59. Jiang H, Wei Z, Ma L, et al. An aqueous dual-ion battery cathode of Mn3O4 via reversible insertion of nitrate. Angew Chem Int Ed 2019;58:5286-91.
60. Zhang F, Wu M, Wang X, et al. Reversible multi-electron redox chemistry of organic salt as anode for high-performance Li-ion/dual-ion batteries. Chem Eng J 2023;457:141335.
61. Huang Z, Hou Y, Wang T, et al. Author correction: manipulating anion intercalation enables a high-voltage aqueous dual ion battery. Nat Commun 2021;12:4885.
62. Sun Z, Zhu K, Liu P, Chen X, Li H, Jiao L. Fluorination treatment of conjugated protonated polyanilines for high-performance sodium dual-ion batteries. Angew Chem Int Ed 2022;61:e202211866.
63. Mu S, Liu Q, Kidkhunthod P, Zhou X, Wang W, Tang Y. Molecular grafting towards high-fraction active nanodots implanted in N-doped carbon for sodium dual-ion batteries. Natl Sci Rev 2021;8:nwaa178.
64. Wu S, Zhang F, Tang Y. A novel calcium-ion battery based on dual-carbon configuration with high working voltage and long cycling life. Adv Sci 2018;5:1701082.
65. Wei C, Gong D, Xie D, Tang Y. The free-standing alloy strategy to improve the electrochemical performance of potassium-based dual-ion batteries. ACS Energy Lett 2021;6:4336-44.
66. He F, Zhou Y, Chen X, et al. A bipolar pyridine-functionalized porphyrin with hybrid charge-storage for dual-ion batteries. Chem Commun 2023;59:2787-90.
67. Yang K, Liu Q, Zheng Y, Yin H, Zhang S, Tang Y. Locally ordered graphitized carbon cathodes for high-capacity dual-ion batteries. Angew Chem Int Ed 2021;60:6326-32.
68. Wang J, Polleux J, Lim J, Dunn B. Pseudocapacitive contributions to electrochemical energy storage in TiO2 (anatase) nanoparticles. J Phys Chem C 2007;111:14925-31.
69. Jiang B, Su Y, Liu R, Sun Z, Wu D. Calcium based all-organic dual-ion batteries with stable low temperature operability. Small 2022;18:e2200049.
70. Lu P, Sun Y, Xiang H, Liang X, Yu Y. 3D Amorphous carbon with controlled porous and disordered structures as a high-rate anode material for sodium-ion batteries. Adv Energy Mater 2018;8:1702434.
71. Tang B, Fang G, Zhou J, et al. Potassium vanadates with stable structure and fast ion diffusion channel as cathode for rechargeable aqueous zinc-ion batteries. Nano Energy 2018;51:579-87.
72. He B, Man P, Zhang Q, et al. All Binder-free electrodes for high-performance wearable aqueous rechargeable sodium-ion batteries. Nanomicro Lett 2019;11:101.
73. Zhou G, An X, Zhou C, Wu Y, Miao Y, Liu T. Highly porous electroactive polyimide-based nanofibrous composite anode for all-organic aqueous ammonium dual-ion batteries. Compos Commun 2020;22:100519.
74. Wu H, Hu T, Chang S, Li L, Yuan W. Sodium-based dual-ion battery based on the organic anode and ionic liquid electrolyte. ACS Appl Mater Interfaces 2021;13:44254-65.
75. Lei X, Zheng Y, Zhang F, Wang Y, Tang Y. Highly stable magnesium-ion-based dual-ion batteries based on insoluble small-molecule organic anode material. Energy Stor Mater 2020;30:34-41.
76. Li Y, Guan Q, Cheng J, Wang B. Flexible high energy density sodium dual-ion battery with long cycle life. Energy Environ Mater 2022;5:1285-93.
77. Park S, Yoo J, Chang B, Ahn E. Novel instrumentation in electrochemical impedance spectroscopy and a full description of an electrochemical system. Pure Appl Chem 2006;78:1069-80.
78. Yang W, Zhou J, Wang S, et al. Freestanding film made by necklace-like N-doped hollow carbon with hierarchical pores for high-performance potassium-ion storage. Energy Environ Sci 2019;12:1605-12.
79. Ma W, Luo L, Huang X, et al. Dihydrophenazine-based conjugated microporous polymer cathodes with enhanced electronic and ionic conductivities for high-performance aluminum dual-ion batteries. Adv Energy Mater 2023;13:2203253.
80. Li Z, Liu J, Niu B, Li J, Kang F. A novel graphite-graphite dual ion battery using an AlCl3-[EMIm]Cl liquid electrolyte. Small 2018;14:e1800745.
81. Tao S, Demir B, Baktash A, et al. Solvent-derived fluorinated secondary interphase for reversible Zn-graphite dual-ion batteries. Angew Chem Int Ed 2023;62:e202307208.
82. Xiang L, Ou X, Wang X, Zhou Z, Li X, Tang Y. Highly concentrated electrolyte towards enhanced energy density and cycling life of dual-ion battery. Angew Chem Int Ed 2020;59:17924-30.
83. Song Z, Zhan H, Zhou Y. Anthraquinone based polymer as high performance cathode material for rechargeable lithium batteries. Chem Commun 2009:448-50.
84. Wu H, Luo S, Li L, Xiao H, Yuan W. A high-capacity dual-ion full battery based on nitrogen-doped carbon nanosphere anode and concentrated electrolyte. Battery Energy 2023;2:20230009.
85. Lin R, Ke C, Chen J, Liu S, Wang J. Asymmetric donor-acceptor molecule-regulated core-shell-solvation electrolyte for high-voltage aqueous batteries. Joule 2022;6:399-417.
86. Liang Z, Gong D, Shang J, et al. Low volume expansion carbon-coated Fe2P4O12 anode material for high-performance sodium dual-ion battery. Energy Stor Mater 2022;53:331-9.
87. Wu N, Yao W, Song X, et al. A calcium-ion hybrid energy storage device with high capacity and long cycling life under room temperature. Adv Energy Mater 2019;9:1803865.
Cite This Article
Export citation file: BibTeX | RIS
OAE Style
Wu H, Luo S, Zheng W, Li L, Fang Y, Yuan W. Metal- and binder-free dual-ion battery based on green synthetic nano-embroidered spherical organic anode and pure ionic liquid electrolyte. Energy Mater 2024;4:400015. http://dx.doi.org/10.20517/energymater.2023.75
AMA Style
Wu H, Luo S, Zheng W, Li L, Fang Y, Yuan W. Metal- and binder-free dual-ion battery based on green synthetic nano-embroidered spherical organic anode and pure ionic liquid electrolyte. Energy Materials. 2024; 4(2): 400015. http://dx.doi.org/10.20517/energymater.2023.75
Chicago/Turabian Style
Wu, Hongzheng, Shenghao Luo, Wen Zheng, Li Li, Yaobing Fang, Wenhui Yuan. 2024. "Metal- and binder-free dual-ion battery based on green synthetic nano-embroidered spherical organic anode and pure ionic liquid electrolyte" Energy Materials. 4, no.2: 400015. http://dx.doi.org/10.20517/energymater.2023.75
ACS Style
Wu, H.; Luo S.; Zheng W.; Li L.; Fang Y.; Yuan W. Metal- and binder-free dual-ion battery based on green synthetic nano-embroidered spherical organic anode and pure ionic liquid electrolyte. Energy Mater. 2024, 4, 400015. http://dx.doi.org/10.20517/energymater.2023.75
About This Article
Special Issue
Copyright
Data & Comments
Data
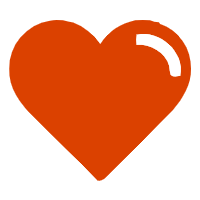

Comments
Comments must be written in English. Spam, offensive content, impersonation, and private information will not be permitted. If any comment is reported and identified as inappropriate content by OAE staff, the comment will be removed without notice. If you have any queries or need any help, please contact us at support@oaepublish.com.